Back to Journals » Journal of Experimental Pharmacology » Volume 16

Viloxazine Increases Extracellular Concentrations of Norepinephrine, Dopamine, and Serotonin in the Rat Prefrontal Cortex at Doses Relevant for the Treatment of Attention-Deficit/Hyperactivity Disorder
Authors Garcia-Olivares J, Yegla B, Bymaster FP, Earnest J, Koch J, Yu C, Rubin J
Received 2 August 2023
Accepted for publication 23 December 2023
Published 16 January 2024 Volume 2024:16 Pages 13—24
DOI https://doi.org/10.2147/JEP.S433524
Checked for plagiarism Yes
Review by Single anonymous peer review
Peer reviewer comments 2
Editor who approved publication: Dr Roger Pinder
Jennie Garcia-Olivares,1 Brittney Yegla,1 Frank P Bymaster,2 Jami Earnest,1 Jennifer Koch,1 Chungping Yu,1 Jonathan Rubin1
1Supernus Pharmaceuticals, Inc., Rockville, MD, USA; 2Bymaster Neuroscience Consulting, Green Cove Springs, FL, USA
Correspondence: Jennie Garcia-Olivares, Supernus Pharmaceuticals, Inc., 9715 Key West Avenue, Rockville, MD, 20850, USA, Fax +1 301-560-5542, Email [email protected]
Background: Viloxazine ER (viloxazine extended-release capsules; Qelbree®), a nonstimulant attention-deficit/hyperactivity disorder (ADHD) treatment, has known activity as a norepinephrine (NE) transporter (NET) inhibitor. In vitro studies have also shown direct pharmacological effects on specific serotonin (5-HT) receptors, but not on the serotonin transporter (SERT). An in vivo microdialysis study in rats showed viloxazine (50 mg/kg i.p.) increased extracellular 5-HT, NE, and dopamine (DA) in the prefrontal cortex (PFC), a key brain region in ADHD pathology. This study evaluated whether these effects occur at clinically relevant concentrations.
Methods: Microdialysis experiments were conducted in freely-moving, Sprague-Dawley rats (males, 8 weeks). Viloxazine (1, 3, 10, 30 mg/kg) was administered intraperitoneally to establish the dose range in rats at which viloxazine plasma concentrations aligned with those of individuals with ADHD administered therapeutic doses of viloxazine ER. Concentrations of unbound viloxazine, NE, 5-HT, DA, and NE and 5-HT metabolites (3,5-dihydroxyphenylglycol [DHPG] and 5-hydroxyindoleacetic acid [5-HIAA]) were measured in PFC interstitial fluid. After identifying a therapeutically relevant dose (30 mg/kg), the experiment was repeated using 30 and 50 mg/kg viloxazine (as 50 mg/kg increased NE, 5-HT, and DA in prior studies).
Results: Viloxazine unbound (free drug) plasma concentrations in rats at 30 mg/kg were comparable to free drug concentrations in individuals with ADHD taking clinically effective doses (based on validated population PK models). Viloxazine 30 mg/kg significantly increased extracellular NE, 5-HT, and DA PFC levels compared to vehicle. Concomitant decreases in DHPG, but not 5-HIAA, support the inhibitory effect of viloxazine on NET but not SERT.
Conclusion: At clinically relevant concentrations, viloxazine increases PFC NE, DA, and 5-HT. Prefrontal augmentation of 5-HT does not appear to result from 5-HT reuptake inhibition but may be related to activation of 5-HT neurons. The potential therapeutic role of serotonergic effects in ADHD treatment merits further exploration.
Plain Language Summary: Viloxazine ER (Qelbree®) is a non-stimulant, FDA-approved treatment for ADHD in children and adults. Viloxazine and other ADHD medications are thought to work by increasing two signaling molecules, called norepinephrine and dopamine, in a brain area called the prefrontal cortex. The prefrontal cortex is important in ADHD as it helps control impulsive behavior, attention, hyperactivity, and learning. A third signaling molecule called serotonin may also be important for ADHD. Previous experiments in rats have shown that viloxazine increases norepinephrine, dopamine, and serotonin in the prefrontal cortex. To better understand how viloxazine increases serotonin and if the increase occurs at the same doses used to treat ADHD, we did another microdialysis study in rats. Our study showed that viloxazine increases norepinephrine, dopamine, and serotonin in the prefrontal cortex at doses used to treat ADHD in humans. We showed that viloxazine increases norepinephrine and dopamine levels in the neuron where these signaling molecules work by blocking the way that they are recycled back into the nerve space. However, viloxazine does not block the serotonin recycling mechanism, meaning viloxazine works differently than selective serotonin reuptake inhibitor (SSRI) medications (often used in depression). Additional studies are needed to determine exactly how viloxazine increases serotonin in the brain and whether this increase plays a role in treating ADHD. These additional studies will also help us understand how viloxazine acts differently than other ADHD medications.
Keywords: viloxazine, microdialysis, prefrontal cortex, serotonin, NET, ADHD
Graphical Abstract:
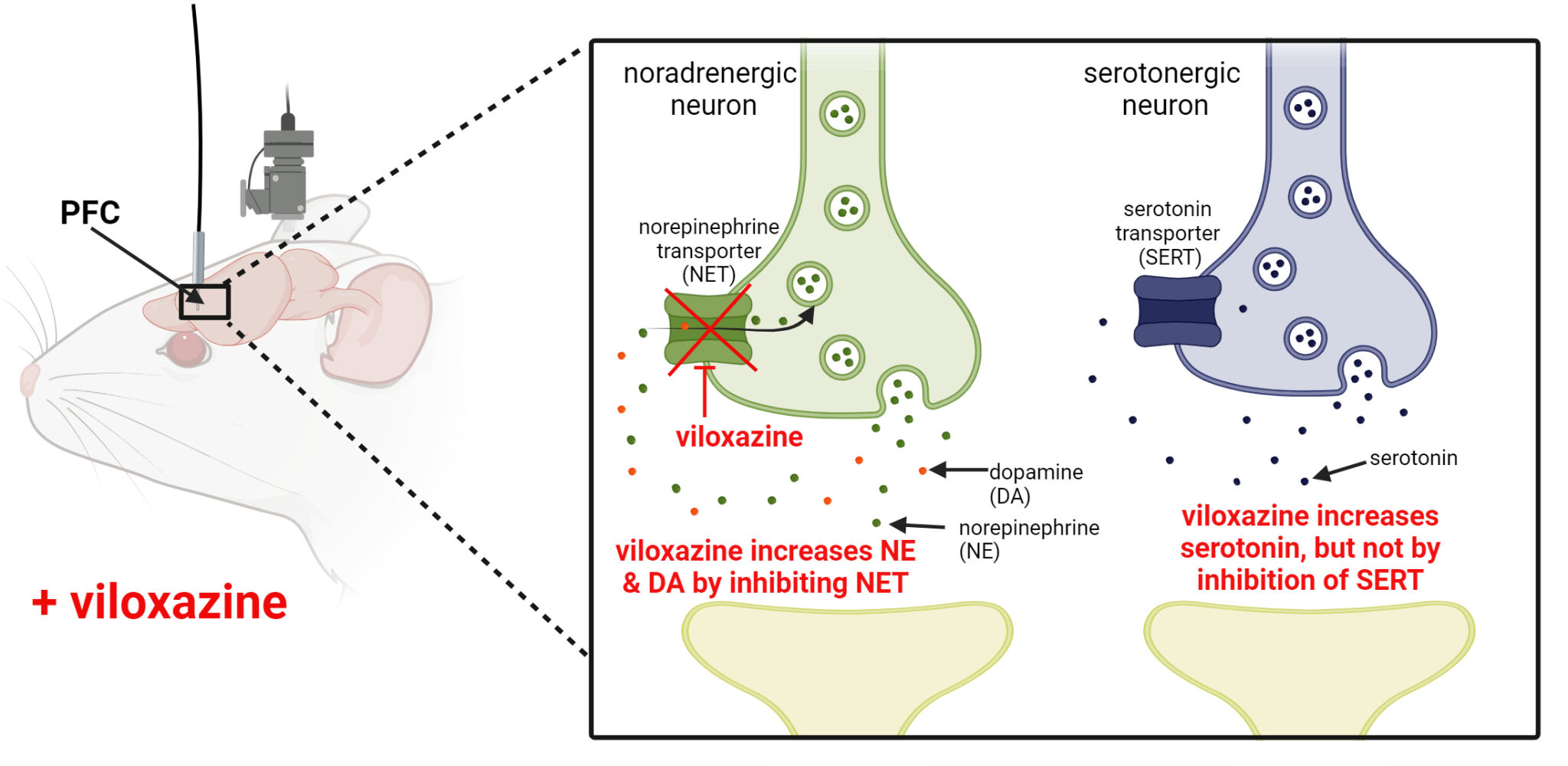
Introduction
Dysregulated dopaminergic and noradrenergic neurotransmission has been widely implicated in the pathophysiology of ADHD based on the fact that most available drugs (including stimulants) act by targeting these neurotransmitter systems.1 A role for serotonin (5-HT) in ADHD has also been delineated; however, due to the superior efficacy of psychostimulants, and the relative ineffectiveness of selective serotonin reuptake inhibitors (SSRI’s) in the treatment of ADHD, the therapeutic potential of targeting the serotonergic system has been less well investigated.2,3
The efficacy of viloxazine in the treatment of ADHD has been attributed to its ability to increase norepinephrine (NE) and dopamine (DA) levels by inhibiting the norepinephrine transporter (NET),4 the major protein responsible for the clearance of catecholamines in the prefrontal cortex (PFC).5–7 In the US, viloxazine ER (viloxazine extended-release capsules; Qelbree®) is approved by the FDA for the treatment of ADHD in children (≥6 years) and adults. This has led to the labeling of viloxazine as a norepinephrine reuptake inhibitor (NRI). However, this narrow description does not consider evidence from early studies (conducted in the 1970s and 1990s) that demonstrated that viloxazine also affects 5-HT transmission, which is not typically associated with NRI’s.5,8–11
More recently, a microdialysis study found that viloxazine increased extracellular levels of NE, DA, and 5-HT in the PFC of freely-moving, male rats.6 The increase in 5-HT found in this study was especially intriguing, as it suggested a potential contribution to the mechanistic action of viloxazine in ADHD; however, this initial study utilized a single dose of viloxazine (50 mg/kg) and did not collect sufficient data to determine whether increases at this dose were clinically relevant.
The aim of the microdialysis study described here was to confirm whether viloxazine, when given at clinically relevant doses, increased extracellular levels of NE, DA, and 5-HT in the rat PFC. Dose-ranging experiments were first conducted to understand the consistency of the neurochemical effect induced by varying concentrations of viloxazine, identify the lowest dose able to elicit an effect on 5-HT neurotransmission in the PFC, and assess for a plateau effect of viloxazine on prefrontal neurotransmitter levels. To assess the clinical relevance of the impact of viloxazine on 5-HT neurotransmission, viloxazine unbound plasma concentrations obtained from rats in these dose-ranging experiments were compared to unbound plasma concentrations in humans treated with viloxazine ER for ADHD. A second set of experiments in this study sought to replicate these effects of viloxazine on 5-HT transmission in the PFC at the clinically relevant dose determined in the first set of experiments in order to fully validate these findings.
Materials and Methods
For a summary of full methodology please see flow chart in Supplementary Figure 1.
Animals
Adult male Sprague-Dawley rats (age, 7–8 weeks; body weight, 300–400 g; Charles River Laboratories, San Francisco, CA, USA) were divided into five treatment groups (N=5–6/group, except for vehicle: N=7). Animals were housed two per cage in polycarbonate cages and acclimated for at least four days prior to study commencement. Animal housing facilities were maintained at a 12 hour/12 hour light/dark cycle, ambient temperature (22+2°C), and ~50% humidity. Animals had access to food and water ad libitum. All experiments were conducted in accordance with protocols (US19002) approved by the Institutional Animal Care and Use Committee of Charles River Laboratories, South San Francisco, and adhered to standards of Guide for the Care and Use of Laboratory Animals.12
Drugs
Viloxazine-HCl was supplied by Supernus Pharmaceuticals, Inc. Viloxazine was reconstituted in 0.9% NaCl (vehicle). A previous microdialysis study utilized a 50 mg/kg dose of viloxazine determined by allometric scaling, which utilizes a correction factor based on body surface area from humans to rats.7 To add on to the results of this previous study, a series of lower doses were chosen for the current study (1, 3, 10, 30 mg/kg) to determine the range of concentrations over which the observed effects may have therapeutic relevance.
Surgery and Microdialysis
As conducted previously,7 rats were anesthetized using isoflurane (2%, 800 mL/min O2), with bupivacaine and carprofen used for analgesia, and were placed in a stereotaxic frame (Kopf Instruments, USA or similar). An I-shaped probe (4 mm polyacrylonitrile membrane) was inserted into the medial PFC (mPFC; coordinates from bregma: +3.4 mm, lateral −0.8 mm, ventral −4.0 mm). Probe placement was verified by gross histology of all animals at the end of the experiment after euthanasia with CO2. Rats with incorrect probe placement were excluded from the statistical analysis.
One day after surgery, the I-shaped probe was connected to a microperfusion pump (Harvard PHD 2000 Syringe pump, Holliston, MA or similar) and perfused with artificial cerebrospinal fluid (aCSF) containing 147 mM NaCl, 3.0 mM KCl, 1.2 mM CaCl2, and 1.2 mM MgCl2 at a flow rate of 1.5 µL/min. Dialysate samples were collected every 30 minutes, with collection of three baseline samples, followed by the administration of specific doses of viloxazine or vehicle (collection continued for 240 minutes post-administration). The rats received intraperitoneal injections of vehicle, 1, 3, 10, or 30 mg/kg viloxazine HCl at a concentration of 5 mL/kg. Dialysate samples were collected into vials containing 15 µL of 0.02 M formic acid (FA) and 0.04% ascorbic acid in ultra-purified H2O. The concentrations of viloxazine, NE, DA, 5-HT, the NE metabolite 3,5-dihydroxyphenylglycol (DHPG), and the 5-HT metabolite 5-hydroxyindoleacetic acid (5-HIAA) were quantified in the dialysate samples using high-performance liquid chromatography (HPLC) coupled with tandem mass spectrometry (MS/MS) detection. Data are expressed as % of baseline (calculated by dividing each post-dose time point by the average basal output time, then multiplying by 100).
Pharmacokinetic Analysis
Blood samples were collected from the lateral tail vein in K2EDTA tubes for 30 minutes (N=3/group) and 90 minutes (N=2/group) post-administration of viloxazine. Blood samples were processed within 30 minutes of collection to isolate the plasma (centrifugation at 2500 g for 10 min at 4°C). The samples were then stored at −80°C until pharmacokinetic analysis. Plasma samples were extracted by protein precipitation using a solution of acetonitrile containing 100 ng/mL glafenine (internal standards). After 5 minutes of incubation at room temperature, the samples were centrifuged for 5 minutes (3100 rpm, 4°C) and the supernatant was diluted 2-fold in ultrapure water. The concentration of viloxazine in plasma samples was determined using HPLC-MS/MS.
Statistical Analysis
Statistical evaluation was performed using SigmaStat v14.0 (Systat Software) and SAS v9.4. The level of statistical significance was set at p<0.05. All data are expressed as mean ± SEM. Data were assessed for normality and a Grubbs’ outlier test was performed. If the Grubbs’ outlier test revealed a significant outlier, the sample was excluded from statistical analysis. The effect of treatment on neurotransmitter and metabolite concentrations was determined using repeated-measures, mixed-effects omnibus ANOVA, with treatment as the independent variable and time as the repeated variable. Significant overall ANOVA’s were followed by Tukey’s post-hoc tests to determine whether there were any significant differences between the groups and time-dependent differences at any time point.
Results
Effect of Viloxazine on Extracellular Monoamine Neurotransmitter and Metabolite Levels in Rat mPFC
To evaluate whether viloxazine had a dose-dependent effect on NE, DA, and 5-HT levels, as well as the NE and 5-HT metabolites, DHPG and 5-HIAA, respectively, in the mPFC, viloxazine (1, 3, 10, and 30 mg/kg i.p.) or vehicle was administered to Sprague-Dawley rats, and the ISF levels of these neurotransmitters and metabolites were measured over time. Twenty-seven rats were included in the analyses due to the loss of one animal from the 30 mg/kg group for incorrect probe placement determined via gross histological inspection.
NE and DHPG
Viloxazine increased extracellular concentrations of NE in the mPFC in a dose-dependent manner, with the peak (545% ± 78% of baseline for the 30 mg/kg dose) occurring 60 minutes after administration (Figure 1A). Repeated measures two-way ANOVA showed significant effects of treatment (F(4,22)=10.34, p<0.0001), time (F(8,22)=25.33, p<0.0001), and the interaction of treatment and time (F(32,22)=3.36, p<0.001) (Table 1). Tukey’s post-hoc analysis revealed that 3, 10, and 30 mg/kg doses of viloxazine significantly increased NE levels (p=0.0335, p=0.0057, and p<0.0001, respectively) compared to vehicle-treated animals. Specifically, in comparison to the vehicle group, the 3 mg/kg dose significantly increased NE levels from 60 minutes to 120 minutes after dosing, the 10 mg/kg dose had significant effects from 30 to 180 minutes, and the 30 mg/kg dose increased NE levels from 30 minutes up to the end of the recording (240 minutes) (Figure 1A). There was a concomitant decrease from baseline in the levels of the NE metabolite, DHPG, in the mPFC (Figure 1B). Statistical analysis showed a significant effect of treatment (F(4,22)=4.51, p=0.0082) and an interaction of treatment and time (F(32,22)=2.04, p=0.0425). Post-hoc analysis showed that, compared to the vehicle group, there was a significant decrease in DHPG levels in animals treated with 10 and 30 mg/kg (p=0.0424 and p=0.0011, respectively). The lowest extracellular levels of DHPG occurred 60 minutes after viloxazine administration at the 10 and 30 mg/kg doses, causing significant decreases (57.2% ± 5.7% of baseline [p=0.0017] and 47.9% ± 9.5% of baseline [p=<0.0001] respectively [Figure 1B]).
![]() |
Table 1 Repeated-Measures, Two-Way ANOVA (Type 3 Fixed Effects). Evaluating the Response of NE, DHPG, DA, 5-HT, and 5-HIAA Levels to Multiple Doses of Viloxazine Over Time |
DA
DA levels did not appear to be significantly altered by viloxazine administration, as there was no significant main effect of treatment or interaction between treatment and time (p=0.64 and 0.08, respectively) (Table 1). The effect of viloxazine (1–30 mg/kg) on DA levels found in this experiment was unexpected and contrary to the findings of a previously published microdialysis experiment by our group, in which a 50 mg/kg dose of viloxazine significantly increased extracellular DA levels in the PFC.6 These time-course experiments were repeated with select doses to verify the effect of viloxazine on DA levels in the PFC (data presented later in the Results section; Figure 4B).
5-HT and 5-HIAA
Viloxazine increased extracellular concentrations of 5-HT in the mPFC, with a peak (197.9% ± 46.0% of baseline levels for the 30 mg/kg dose) occurring 90 minutes after administration (Figure 2A). A repeated-measures two-way ANOVA of 5-HT levels following the administration of viloxazine showed a significant effect of treatment (F(4, 21)=3.71; p=0.0195), time (F(8,21)=28.17); p<0.0001), and a significant interaction between treatment and time (F(32,21)=2.26, p=0.0268) (Table 1). Tukey’s post-hoc analysis revealed an overall significant effect for the 30 mg/kg dose of viloxazine compared to vehicle, with significant increases in 5-HT levels specifically from 30 to 180 minutes (with the exception of 150 minutes) post-viloxazine administration (Figure 2A). As shown in Figure 2B, the levels of the 5-HT metabolite, 5-HIAA, were not affected by viloxazine treatment (F(4.22)= 0.62, p=0.6500), and there was no interaction between treatment and time (F(32,22)=2.26, p=0.1047) (Table 1).
Extracellular Viloxazine Levels in Rat mPFC Over Time
Viloxazine levels were quantifiable in ISF at all tested doses (1, 3, 10, and 30 mg/kg) from 30 to 240 minutes (Supplementary Figure 2). All four doses reached their peak in ISF 60 minutes after dosing (i.p.) (Tmax=60 min). At Tmax, viloxazine ISF levels were 0.088 µM ± 0.003, 0.184 µM ± 0.057, 1.128 µM ± 0.413, 3.125 µM ± 1.344 for 1, 3, 10, and 30 mg/kg doses, respectively.
Determination of a Clinically Relevant Dose of Viloxazine in Rats
The clinical relevance of the viloxazine doses used in this study was determined by comparing plasma concentrations of unbound viloxazine (VLXunbound) in rats obtained during the study to the range of unbound plasma concentrations estimated from approved doses of viloxazine extended-release in children, adolescents, and adults with ADHD (fraction unbound in plasma: rat=40%; human=21%) (Supernus data on file).13 Average unbound plasma concentrations were obtained from pediatric and adult population pharmacokinetic models developed using steady-state plasma concentration data from over 500 Phase 3 clinical trial participants who received viloxazine in the extended-release formulation (Figure 3A) (Supernus data on file).14 The clinically effective range of unbound viloxazine in plasma was considered to be from 0.4 µM, which is the lower confidence interval of the minimum approved, effective viloxazine ER dose in children (100 mg/day), to 3.6 µM, which is the higher confidence interval for the maximum approved, effective dose of viloxazine ER in children (400 mg/day) (Figure 3A). This “clinically relevant” range was applied to rat VLXunbound plasma concentrations obtained 30- and 90-minutes post-administration (Figure 3B). Plasma was not collected at 60 minutes post-dosing; therefore, this range could not be applied to the Cmax. Using this approach, the 10 and 30 mg/kg doses (i.p.) in rats were identified as clinically relevant, as they reached VLXunbound concentrations that overlapped with the clinically relevant concentration range in human patients (Figure 3B).
The first experiments in this study demonstrated that at the clinically relevant dose of viloxazine (30 mg/kg), NE and 5-HT levels were significantly increased in the mPFC compared to vehicle (Figure 1A and 2A). To further substantiate these findings and verify their unexpected effects on DA, additional time course experiments were performed.
Effect of 30 and 50 mg/kg Doses of Viloxazine on Extracellular Monoamine Neurotransmitter Levels in Rat mPFC
To evaluate the effects of a clinically relevant dose of viloxazine (30 mg/kg) and a dose of viloxazine extensively characterized in a previous report (50 mg/kg) on NE, DA, and 5-HT in the rat mPFC, 30 and 50 mg/kg doses of viloxazine were administered to Sprague-Dawley rats (i.p.), and neurotransmitter concentrations in the PFC were measured over time. These time-course microdialysis experiments were conducted as described in the methods section above, except that: (1) only two doses of viloxazine and vehicle were utilized, (2) a separate cohort of 19 male, Sprague-Dawley rats were divided into three treatment groups (N=6–7/group; 30 mg/kg, 50 mg/kg, vehicle), (3) DHPG and 5-HIAA levels were not quantified, but the DA metabolite, DOPAC, levels were (4) plasma samples were not collected, and (5) the I-shaped probe consisted of a 2 mm polyacrylonitrile membrane instead of a 4 mm membrane.
Both the 30 and 50 mg/kg doses of viloxazine increased extracellular monoamine neurotransmitter levels in the mPFC, with the highest extracellular NE, DA, and 5-HT concentrations occurring 60 minutes after administration (30 mg/kg: 365% ± 15%, 182% ± 10%, 302 ± 36%; 50 mg/kg: 473% ± 35%, 241% ± 32%, 356% ± 49% of baseline, respectively) (Figure 4). For all three neurotransmitters, there were significant overall effects of treatment and time as well as a significant interaction between the effect of treatment and time (Table 2). Post-hoc analyses revealed significant effects of both doses on NE and DA levels compared to vehicle from 30 to 180 minutes post-dosing (Figure 4A and B), and 5-HT levels were significantly increased compared to vehicle at selected time points from 60 to 180 minutes post-dosing (Figure 4C). A one-way ANOVA of the area under the curve (AUC) also revealed a significant overall effect of treatment for NE, DA, and 5-HT (F(2,16)=49.46, p<0.0001; F(2,16)=19.33, p<0.0001; F(2,16)=7.698, p=0.0045, respectively), and post-hoc tests revealed significant differences from vehicle for 30 and 50 mg/kg doses of viloxazine for NE (p<0.0001 for both), DA (p=0.0008 and p<0.0001, respectively), and 5-HT (p<0.0152 and p=0.0062, respectively) (Figure 4). DOPAC levels were not quantifiable; therefore, the data are not shown.
![]() |
Table 2 Repeated-Measures, Two-Way ANOVA (Type 3 Fixed Effects). Evaluating the Response of NE, DA, and 5-HT Levels to 30 and 50 mg/kg of Viloxazine Over Time |
Concentration-Related Pharmacodynamic Effect of Viloxazine on Neurotransmission in the PFC
The concentration of viloxazine in the ISF over time mirrored the percentage increase in NE, DA, and 5-HT levels compared to baseline in the ISF (Figure 5A). The increase in 5-HT levels compared to baseline most closely resembled the pattern of viloxazine increase in the ISF, with both reaching their peak concentrations (Cmax) 60 minutes after viloxazine administration and then decreasing sharply at 90 minutes (Figure 5A).
Figure 5B shows an overall summary of PK/PD effects in all experiments in this study. Overall, the relative levels of 5-HT in the PFC were correlated with free-drug viloxazine concentrations in the ISF, demonstrating a clear PK/PD relationship (Figure 5B).
Discussion
Although viloxazine is often described as an NRI, previous experiments have suggested that serotonergic effects may also be relevant to its therapeutic actions.5,6,11 Our study supports this premise and shows that administration of viloxazine results in an increase in 5-HT neurotransmission (as well as NE and DA) in the PFC of healthy animals, and that these increases are concentration- and time-dependent and occur at viloxazine plasma concentrations that are clinically relevant for the treatment of ADHD. The lack of a coincident decrease in 5-HIAA levels suggests that, unlike SSRI’s which block SERT, viloxazine increases 5-HT by an alternate mechanism.
By administering multiple doses of viloxazine (1, 3, 10, or 30 mg/kg) to rats, we were able to establish that there is a concentration-related pharmacodynamic effect of viloxazine on prefrontal neurotransmitter levels, while simultaneously obtaining plasma concentrations to determine a clinically relevant concentration range by comparing unbound viloxazine concentrations in these animals to those seen in children and adults taking viloxazine ER for ADHD. We found that the 10 and 30 mg/kg doses administered to rats produced unbound viloxazine plasma concentrations that were consistent with the clinically relevant range expected during ADHD treatment (Figure 3B). Moreover, the 30 mg/kg dose significantly increased NE, DA, and 5-HT levels in the extracellular fluid of the PFC compared to both vehicle treatment and baseline (Figures 1, 2, and 4). The effects of 30 mg/kg viloxazine on prefrontal 5-HT were confirmed in a second set of microdialysis experiments, adding further confidence in our findings. Increased 5-HT, NE, and DA levels in the PFC were directly correlated with ISF levels of viloxazine in the brain at 30 mg/kg. 5-HT most closely followed the pattern of increased viloxazine ISF levels, with both peaking at 60 minutes post-administration and sharply falling at 90 minutes post-administration (Figure 5A). Therefore, changes in neurotransmitter levels, particularly those of 5-HT, can be directly ascribed to the pharmacological effects of viloxazine in the PFC. Overall, these results show a clear PK/PD relationship with relative levels of 5-HT in the PFC correlating with free-drug viloxazine concentrations in the ISF (Figure 5B). Extrapolating from this data, we would expect that at clinically relevant viloxazine (unbound) concentrations (Adult 600 mg/day Cave=2.4 µM ± 0.9 µM, Children 400 mg/kg Cave= 2.5 µM ± 1.1 µM), the level of increased 5-HT in the PFC of humans would theoretically be ≥200%, comparable to levels obtained with SSRI’s (185–290%).15
Quantification of the NE and 5-HT metabolites, DHPG and 5-HIAA, allowed us to determine whether the observed increases in NE and 5-HT were due to viloxazine-induced blockade of NET and SERT, respectively. These insights into the mechanism of action of viloxazine enable us to compare the effects of viloxazine to the known therapeutic actions of other drugs with these mechanisms. Viloxazine-induced increases in extracellular NE levels were accompanied by concomitant decreases in extracellular DHPG levels (Figure 1). This supports the interpretation that viloxazine increases NE (and DA) in the PFC by inhibiting NET,4 in line with previous observations of its moderate inhibitory activity of NET (IC50=0.3 µM) in rat synaptosome preparations,6 and characterization as a NRI. In contrast, viloxazine-induced increases in extracellular 5-HT levels in the PFC were not accompanied by alterations in 5-HIAA levels (Figure 2).15 This observation indicates that viloxazine does not increase 5-HT by inhibiting SERT, but rather by some other mechanism. Viloxazine most likely elicits this effect by directly engaging serotonin receptors, in line with the identification of its activity at specific 5-HT receptors in our prior study6 and early studies hypothesizing that the potentiation of 5-HT behavioral effects in mice was likely due to the action of viloxazine on postsynaptic 5-HT receptors.5,11 The lack of a coincident effect on 5-HIAA also demonstrates that, despite its prior success as an antidepressant, viloxazine does not act as an SSRI to inhibit SERT. This is in contrast to SSRI's, such as citalopram and sertraline, which increase extracellular 5-HT and decrease extracellular 5-HIAA levels in the frontal cortex.15,16
Viloxazine’s ability to increase prefrontal 5-HT levels is not unique among ADHD treatments. Similar effects have been observed in microdialysis studies with amphetamines, which are known to be highly effective for ADHD,17 but not with methylphenidate or other NRI’s such as atomoxetine or reboxetine.8–10 However, in a recent study, quantification of 5-HT levels in PFC homogenates of male albino Wistar rats administered repeated doses of methylphenidate, associated with memory enhancement and therefore potentially therapeutic for the treatment of ADHD, found significantly increased levels of 5-HT versus vehicle-treated rats.18 It should also be noted that atomoxetine has been shown to bind SERT;7,19 however, SERT inhibition occurred mostly in the dorsal raphe, not the PFC20,21 and the therapeutic relevance of SERT-specific 5-HT increases is unknown for ADHD.
While the role of 5-HT transmission in ADHD is not completely understood, many studies have suggested that 5-HT deficits can exacerbate ADHD symptoms, in particular impulsivity and hyperactivity, and associated behaviors such as aggression, whereas enhancement of 5-HT may reduce these behaviors.22–26 Although increasing levels of 5-HT are in itself insufficient to adequately treat ADHD (as demonstrated by lack-luster effects of SSRI’s for this condition),2 increasing 5-HT along with NE and DA could increase the treatment efficacy of therapies for ADHD. Regardless, the unique ability of viloxazine to exert serotonergic effects at clinically used doses in ADHD sets it apart from other NRI’s, such as atomoxetine and reboxetine, suggesting that characterization simply as a NRI is insufficient and needs to be broadened.
Limitations
The limitations of this study include the differential DA responses between the various sets of microdialysis experiments in this study and our previous study. The lack of an observable dopaminergic response in the first dose-response experiments of this study was surprising (Table 1), both because DA increases in the PFC have been well documented with NET inhibition and increases in DA were seen in earlier studies, including our previous microdialysis study.6,27 NET has relatively poor selectivity and is able to clear both NE and DA, particularly in the PFC, where DAT is relatively lacking compared to other brain regions.4,28–30 The second set of microdialysis experiments in this study was conducted to reconcile the unexpected results of the first set of experiments in this study with our previously published work (conducted with a 50 mg/kg dose), as well as to confirm the 5-HT effect. These subsequent experiments showed that both 30 and 50 mg/kg viloxazine doses significantly increased NE, 5-HT, and DA over time relative to the vehicle (Figure 4), leading us to conclude that confounding factors, such as longer probe length or individual physiology, may have influenced the lack of DA alterations in the initial experiments of this study. Another limitation of this study is the potential for differences in CNS penetration of viloxazine unbound concentrations in humans relative to rats. We found only one published study31 comparing human blood and CSF concentrations of immediate-release viloxazine, however the data reporting did not allow for easy extrapolation to our experiment. We instead compared unbound drug concentrations in human plasma to unbound drug concentrations in rat plasma samples. Another limitation was that rat plasma concentrations were only obtained from a portion of the viloxazine-treated rats and only at 30 or 90 minutes following each dose, resulting in a potentially incomplete understanding of the relationship between plasma and ISF concentrations over time. Altogether, the limited human CSF data, the inability to collect ISF samples in humans, and the low number of plasma samples we obtained in rats impact our confidence in the direct translation of our preclinical findings to humans taking recommended doses of viloxazine ER for ADHD. Finally, this study was conducted only in male rats, therefore we are unsure if sex differences affect viloxazine induced increases in extracellular neurotransmitter levels in the PFC.
Future Perspectives
The mechanism by which viloxazine increases 5-HT in the PFC and the potential role of 5-HT in the treatment of the core symptoms of ADHD deserve further exploration. Conducting follow-up studies would help to further determine the contribution of increased 5-HT to the efficacy of viloxazine and which subtypes of patients could most benefit from this treatment.
Conclusions
Overall, this study clearly demonstrates that viloxazine can affect 5-HT signaling in the PFC at clinically relevant doses for the treatment of ADHD. These data suggest that, in addition to its known noradrenergic effects as an NRI, the activity of viloxazine on serotonergic PFC transmission may contribute to its established therapeutic efficacy in ADHD.
Acknowledgments
Kobby Asubonteng for assistance with statistical analysis.
Funding
This study was fully funded by Supernus Pharmaceuticals, Inc.
Disclosure
JG-O, BY, JE, JK, CY are employees of Supernus Pharmaceuticals, Inc. FB is an employee of Bymaster Neuroscience Consulting and a consultant for Supernus Pharmaceuticals, Inc. JR is an employee of Supernus Pharmaceuticals, Inc. and reports stock options and grants from Supernus Pharmaceuticals, Inc.
References
1. Sonuga-Barke EJS, Becker SP, Bölte S, et al. Annual research review: perspectives on progress in ADHD science – from characterization to cause. J Child Psychol Psychiatry. 2023;64(4):506–532. doi:10.1111/jcpp.13696
2. Popper CW. Antidepressants in the treatment of attention-deficit/hyperactivity disorder. J Clin Psychiatry. 1997;58(14):14–29.
3. Otasowie J, Castells X, Ehimare UP, Smith CH. Tricyclic antidepressants for attention deficit hyperactivity disorder (ADHD) in children and adolescents. Cochrane Database Syst Rev. 2014;(9):Cd006997. doi:10.1002/14651858.CD006997.pub2
4. Morón JA, Brockington A, Wise RA, Rocha BA, Hope BT. Dopamine uptake through the norepinephrine transporter in brain regions with low levels of the dopamine transporter: evidence from knock-out mouse lines. J Neurosci. 2002;22(2):389–395. doi:10.1523/jneurosci.22-02-00389.2002
5. Carlier PR, Lo MM, Lo PC, et al. Synthesis of a potent wide-spectrum serotonin-, norepinephrine-, dopamine-reuptake inhibitor (SNDRI) and a species-selective dopamine-reuptake inhibitor based on the gamma-amino alcohol functional group. Bioorg Med Chem Lett. 1998;8(5):487–492. doi:10.1016/s0960-894x(98)00062-6
6. Yu C, Garcia-Olivares J, Candler S, Schwabe S, Maletic V. New insights into the mechanism of action of viloxazine: serotonin and norepinephrine modulating properties. J Exp Pharmacol. 2020;12:285–300. doi:10.2147/jep.S256586
7. Tatsumi M, Groshan K, Blakely RD, Richelson E. Pharmacological profile of antidepressants and related compounds at human monoamine transporters. Eur J Pharmacol. 1997;340(2–3):249–258. doi:10.1016/s0014-2999(97)01393-9
8. Rowley HL, Kulkarni RS, Gosden J, Brammer RJ, Hackett D, Heal DJ. Differences in the neurochemical and behavioural profiles of lisdexamfetamine methylphenidate and modafinil revealed by simultaneous dual-probe microdialysis and locomotor activity measurements in freely-moving rats. J Psychopharmacol. 2014;28(3):254–269. doi:10.1177/0269881113513850
9. Bymaster FP, Katner JS, Nelson DL, et al. Atomoxetine increases extracellular levels of norepinephrine and dopamine in prefrontal cortex of rat: a potential mechanism for efficacy in attention deficit/hyperactivity disorder. Neuropsychopharmacology. 2002;27(5):699–711. doi:10.1016/s0893-133x(02)00346-9
10. Koda K, Ago Y, Cong Y, Kita Y, Takuma K, Matsuda T. Effects of acute and chronic administration of atomoxetine and methylphenidate on extracellular levels of noradrenaline, dopamine and serotonin in the prefrontal cortex and striatum of mice. J Neurochem. 2010;114(1):259–270. doi:10.1111/j.1471-4159.2010.06750.x
11. Lippman W, Pugsley TA. Effects of viloxazine, an antidepressant agent, on biogenic amine uptake mechanisms and related activities. Can J Physiol Pharmacol. 1976;54(4):494–509. doi:10.1139/y76-069
12. Institute of Laboratory Animal Resources (US). Committee for the Update of the Guide for the Care and Use of Laboratory Animals IfLAR, Division on Earth and Life Studies, and National Research Council.
13. Supernus Pharmaceuticals, Inc. Qelbree [package insert]. Supernus Pharmaceuticals, Inc; 2022.
14. Nasser A, Gomeni R, Wang Z, et al. Population pharmacokinetics of viloxazine extended-release capsules in pediatric subjects with attention deficit/hyperactivity disorder. J Clin Pharmacol. 2021;61(12):1626–1637. doi:10.1002/jcph.1940
15. Stenfors C, Ross SB. Changes in extracellular 5-HIAA concentrations as measured by in vivo microdialysis technique in relation to changes in 5-HT release. Psychopharmacology. 2004;172(2):119–128. doi:10.1007/s00213-003-1736-z
16. Mikail HG, Dalla C, Kokras N, Kafetzopoulos V, Papadopoulou-Daifoti Z. Sertraline behavioral response associates closer and dose-dependently with cortical rather than hippocampal serotonergic activity in the rat forced swim stress. Physiol Behav. 2012;107(2):201–206. doi:10.1016/j.physbeh.2012.06.016
17. Cortese S, Adamo N, Del Giovane C, et al. Comparative efficacy and tolerability of medications for attention-deficit hyperactivity disorder in children, adolescents, and adults: a systematic review and network meta-analysis. Lancet Psychiatry. 2018;5(9):727–738. doi:10.1016/s2215-0366(18)30269-4
18. Salman T, Afroz R, Nawaz S, Mahmood K, Haleem DJ, Zarina S. Differential effects of memory enhancing and impairing doses of methylphenidate on serotonin metabolism and 5-HT1A, GABA, glutamate receptor expression in the rat prefrontal cortex. Biochimie. 2021;191:51–61. doi:10.1016/j.biochi.2021.08.009
19. Ding YS, Naganawa M, Gallezot JD, et al. Clinical doses of atomoxetine significantly occupy both norepinephrine and serotonin transports: implications on treatment of depression and ADHD. NeuroImage. 2014;86:164–171. doi:10.1016/j.neuroimage.2013.08.001
20. Charnay Y, Léger L. Brain serotonergic circuitries. Dialogues Clin Neurosci. 2010;12(4):471–487. doi:10.31887/DCNS.2010.12.4/ycharnay
21. Dankoski EC, Carroll S, Wightman RM. Acute selective serotonin reuptake inhibitors regulate the dorsal raphe nucleus causing amplification of terminal serotonin release. J Neurochem. 2016;136(6):1131–1141. doi:10.1111/jnc.13528
22. Angoa-Pérez M, Kane MJ, Briggs DI, et al. Genetic depletion of brain 5HT reveals a common molecular pathway mediating compulsivity and impulsivity. J Neurochem. 2012;121(6):974–984. doi:10.1111/j.1471-4159.2012.07739.x
23. Moeller FG, Dougherty DM, Swann AC, Collins D, Davis CM, Cherek DR. Tryptophan depletion and aggressive responding in healthy males. Psychopharmacology. 1996;126(2):97–103. doi:10.1007/bf02246343
24. Eagle DM, Lehmann O, Theobald DEH, et al. Serotonin depletion impairs waiting but not stop-signal reaction time in rats: implications for theories of the role of 5-HT in behavioral inhibition. Neuropsychopharmacology. 2009;34(5):1311–1321. doi:10.1038/npp.2008.202
25. Chantiluke K, Barrett N, Giampietro V, et al. Inverse fluoxetine effects on inhibitory brain activation in non-comorbid boys with ADHD and with ASD. Psychopharmacology. 2015;232(12):2071–2082. doi:10.1007/s00213-014-3837-2
26. Banerjee E, Nandagopal K. Does serotonin deficit mediate susceptibility to ADHD? Neurochem Int. 2015;82:52–68. doi:10.1016/j.neuint.2015.02.001
27. Martinez C, Dominiak P, Kees F, Grobecker H. Inhibition of monoamine oxidase by viloxazine in rats. Arzneimittelforschung. 1986;36(5):800–803.
28. Swanson CJ, Perry KW, Koch-Krueger S, Katner J, Svensson KA, Bymaster FP. Effect of the attention deficit/hyperactivity disorder drug atomoxetine on extracellular concentrations of norepinephrine and dopamine in several brain regions of the rat. Neuropharmacology. 2006;50(6):755–760. doi:10.1016/j.neuropharm.2005.11.022
29. Martiniova L, Cleary S, Lai EW, et al. Usefulness of [18F]-DA and [18F]-DOPA for PET imaging in a mouse model of pheochromocytoma. Nucl Med Biol. 2012;39(2):215–226. doi:10.1016/j.nucmedbio.2011.07.007
30. Harvey RC, Sen S, Deaciuc A, Dwoskin LP, Kantak KM. Methylphenidate treatment in adolescent rats with an attention deficit/hyperactivity disorder phenotype: cocaine addiction vulnerability and dopamine transporter function. Neuropsychopharmacology. 2011;36(4):837–847. doi:10.1038/npp.2010.223
31. Elwan O, Adam HK. Relationship between blood and cerebrospinal levels of the antidepressant agent viloxazine. Eur J Clin Pharmacol. 1980;17(3):179–182. doi:10.1007/bf00561897
© 2024 The Author(s). This work is published and licensed by Dove Medical Press Limited. The full terms of this license are available at https://www.dovepress.com/terms.php and incorporate the Creative Commons Attribution - Non Commercial (unported, v3.0) License.
By accessing the work you hereby accept the Terms. Non-commercial uses of the work are permitted without any further permission from Dove Medical Press Limited, provided the work is properly attributed. For permission for commercial use of this work, please see paragraphs 4.2 and 5 of our Terms.