Back to Journals » International Journal of Nanomedicine » Volume 19
How Precise are Nanomedicines in Overcoming the Blood–Brain Barrier? A Comprehensive Review of the Literature
Authors Mohapatra P, Gopikrishnan M, Doss C GP, Chandrasekaran N
Received 20 October 2023
Accepted for publication 21 December 2023
Published 8 March 2024 Volume 2024:19 Pages 2441—2467
DOI https://doi.org/10.2147/IJN.S442520
Checked for plagiarism Yes
Review by Single anonymous peer review
Peer reviewer comments 3
Editor who approved publication: Dr Farooq A. Shiekh
Priyadarshini Mohapatra,1 Mohanraj Gopikrishnan,2 George Priya Doss C,2 Natarajan Chandrasekaran1
1Centre for Nanobiotechnology, Vellore Institute of Technology, Vellore, TN, 632014, India; 2Department of Integrative Biology, School of Bioscience and Technology, Vellore Institute of Technology, Vellore, TN, 632014, India
Correspondence: Natarajan Chandrasekaran, Centre for Nanobiotechnology, Vellore Institute of Technology, Vellore, TN, 632014, India, Tel +91 416 2202624, Fax +914162243092, Email [email protected]; [email protected]
Abstract: New nanotechnology strategies for enhancing drug delivery in brain disorders have recently received increasing attention from drug designers. The treatment of neurological conditions, including brain tumors, stroke, Parkinson’s Disease (PD), and Alzheimer’s disease (AD), may be greatly influenced by nanotechnology. Numerous studies on neurodegeneration have demonstrated the effective application of nanomaterials in the treatment of brain illnesses. Nanocarriers (NCs) have made it easier to deliver drugs precisely to where they are needed. Thus, the most effective use of nanomaterials is in the treatment of various brain diseases, as this amplifies the overall impact of medication and emphasizes the significance of nanotherapeutics through gene therapy, enzyme replacement therapy, and blood-barrier mechanisms. Recent advances in nanotechnology have led to the development of multifunctional nanotherapeutic agents, a promising treatment for brain disorders. This novel method reduces the side effects and improves treatment outcomes. This review critically assesses efficient nano-based systems in light of obstacles and outstanding achievements. Nanocarriers that transfer medications across the blood-brain barrier and nano-assisted therapies, including nano-immunotherapy, nano-gene therapy, nano enzyme replacement therapy, scaffolds, and 3D to 6D printing, have been widely explored for the treatment of brain disorders. This study aimed to evaluate existing literature regarding the use of nanotechnology in the development of drug delivery systems that can penetrate the blood-brain barrier (BBB) and deliver therapeutic agents to treat various brain disorders.
Keywords: drug delivery, nanomedicine, nano-therapeutics, nanocarriers, blood-brain barrier
Graphical Abstract:
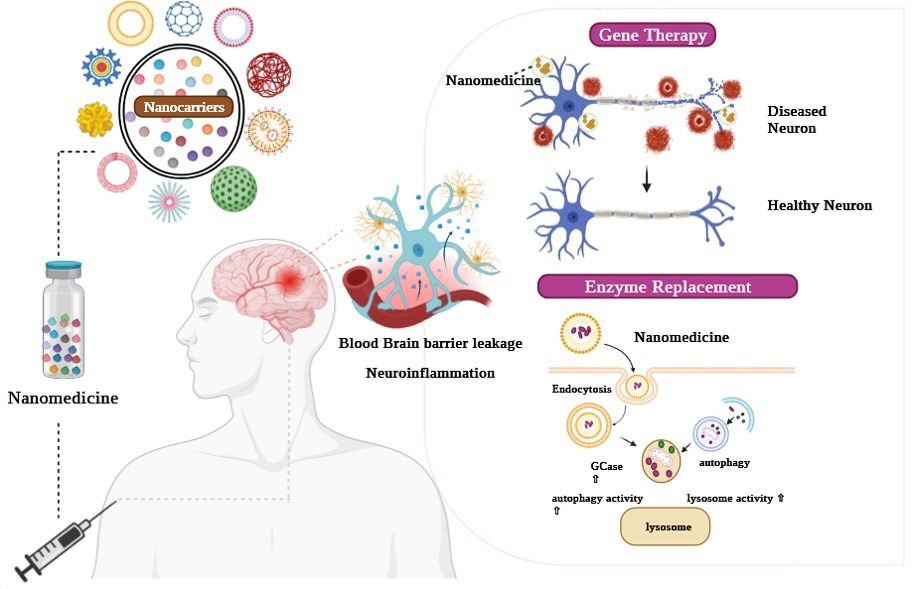
Introduction
The incidence and prevalence of central nervous system (CNS) illnesses are progressively rising, posing serious threats to human life, and increasing socioeconomic expenses associated with providing medicinal treatment.1 The number of disability-adjusted life-years (DSLYs), a crucial indicator of the burden of neurological diseases, is rising.2 In India, the incidence of neurological disorders is growing alarmingly—stroke (37.9%), headache disorders (17.5%), epilepsy (11.3%), cerebral palsy (5.7%), and encephalitis (5.3%).3 The two categories of CNS diseases that affect the brain’s neurological functions are acute brain injury and neurodegenerative disorders. The most common brain injuries are stroke, cerebral ischemia, and epilepsy, whereas chronic injuries include Alzheimer’s Disease (AD), Parkinson’s Disease (PD), and Huntington’s disease.4 Neurological disorders involve the progressive deterioration of neuronal structure and function, often leading to neural death.5 These disorders affect the central and peripheral nervous systems, including the brain, spinal cord, cranial and peripheral nerves, nerve roots, autonomic nervous system (ANS), neuromuscular junctions, and muscles. These disorders include cerebrovascular diseases, PD, AD, Huntington’s disease, amyotrophic lateral sclerosis, multiple sclerosis, brain tumors, stroke, neuroinfections, autism spectrum disorders, and schizophrenia.6,7 The presence of peripheral and blood–brain barriers (BBB) makes it more challenging to deliver drug molecules to target sites in the CNS.8 The most delicate and intricate organs in the human brain are shielded by the BBB. It protects brain neurons against destructive and poisonous substances in the blood and disturbs drug penetration into brain tissues.9 The brain is differentiated from the other parts of the body by strong barriers that prevent the transfer of drug molecules to the CNS. These limitations necessitate the development of new therapeutic approaches to treat brain diseases. Figure 1 introduces innovative nanoparticle (NP)-based brain medication delivery methods.10
Recently, nanotechnology has played a vital role in numerous disease states and has had more therapeutic success. Studies have shown that nanotechnology is more effective than current therapeutics (Figure 1). Some of the nanotechnology applications include acting as (NCs) for drug delivery systems,11 nano-based therapeutic approaches, nano-based stem cell therapy, nano gene therapy,12 nano enzyme replacement therapy, and personalized nanomedicine for future therapeutics. Gene therapy has a significant impact on the neurological sciences. It suppresses or replaces the abnormal cells in their genetic elements and acts as a nano gene carrier in several neurological disorders. Owing to the BBB in patients with glioblastoma, traditional intracerebral injections can result in neurological damage and poor transgene signatures. To address these challenges, studies have explored the application of gene therapy by designing non-viral gene delivery systems for treating angiogenesis for effective gene therapy without damaging brain tissues.13
To bolster these endeavors, the incorporation of nano-based gene therapy has emerged as a promising approach for treating various cancerous cell types. Enzyme replacement therapy (ERT) is a promising treatment option. It replaces or supplements enzymes in patients with enzyme deficiencies or malnutrition. The enzyme was encapsulated in biocompatible nanosubstances, including micelles, liposomes, polymers, and lipid-based NPs. Figure 2 briefly overviews the ideal NCs qualities for delivering drugs to the brain.1 With the help of a NC, we can overcome the drawbacks of ERT, including immunological response and degradation,14 and preserve enzyme functions and activity (Figure 2). This is an effective pharmacological response that controls the release.15–19 NCs are precise and accurate in drug release, exactly targeting drug delivery to the brain. The characteristic of NCs invloves, biocompatibility with diverse substances, protecting the brain cells non-invasively, stability in the bloodstream, the capacity of a molecule to pass through vessels, an extended time for blood circulation, safe, and decomposable.17–19
Additionally, targeting molecules such as antibodies, peptides, and aptamers were loaded with NCs that penetrate the BBB and blood-ocular barriers and directly reached the target sites. This review aims to highlight new findings in the development of nano-based gene therapy and nano-based enzyme replacement therapies for their potential use in the treatment of neurological diseases, especially brain tumors and other neurodegenerative disorders, as well as ongoing clinical trials and other therapeutic challenges in the development of nano-based therapeutic solutions from bench to bedside for overcoming neurodegenerative diseases.
How Critical are Neurological Disorders and Their Treatment?
Neurological illnesses are the biggest threat to the healthcare system, especially in older people. They suffer from neurological issues such as neuroinflammation, neurodegeneration, and cognitive dysfunction. Treatment strategies are often palliative and inadequate to address the disorders.20 The most prevalent neurological disorders are AD, PD, stroke, and brain cancer, which are the most challenging conditions for designing potent therapeutics.
Alzheimer’s Disease
The most common form of dementia is AD, which affects nearly 50 million individuals were affected globally.21,22 Cognitive deficiencies are treated through the intranasal delivery of medicines that target neurotransmitters or enzyme regulations.23 The Food and Drug Administration (FDA) has only permitted four therapies for AD, and the targets focus on various elements of the two primary molecular processes involved in their accumulation of Aβ peptide and neurofibrillary tangles (NFT) of p-tau protein.24 However, these drugs show a significant increase in therapeutic failure due to their inability to penetrate the membranes of neuronal cells, instability, neurotoxicity, and other pharmacokinetic and pharmacodynamic properties.22 To overcome this problem, NPs, such as lipids, polymers, and metals, act as drug delivery systems and even diagnose diseases. These NPs act as drug delivery systems to effectively treat chronic CNS disorders. It is difficult for these drugs to reach organs such as the CNS, especially the BBB region, which must penetrate and regulate the release of drugs.25
Parkinson’s Disease
PD is the second most common neurological degenerative disorder that worsens over time and causes tremors, muscular rigidity, uneven gait, and issues with balance and coordination. Hereditary and nongenetic factors trigger PD, and age is considered the primary factor.26,27 Numerous processes are crucial in developing PD, including the misfold and aggregated α-synuclein, mitochondrial dysfunction, dysfunction of protein clearance mechanisms, ubiquitination of proteasome region, and autophagy of lysozyme and neurological inflammation.27 Similarly, Lewy bodies act as the development of abnormal proteins such as α-synuclein in the neuronal cell bodies affected and featured in PD.28 Owing to BBB restrictions, current drug delivery to the brain cannot restore or maintain the cytoarchitecture acceptably.29 Numerous nanodelivery systems, such as nano-phytobioactive substances, have shown promise in PD treatment. The use of solid lipid NPs, nanolipid carriers, and nanoliposomes has further enhanced the permeability, solubility, and stability of substances for delivery to the target region.30
Stroke
Stroke is a fatal and severely disabling brain condition. Most cases of stroke are ischemic. Currently, no effective treatments are available in the clinic, partly because it is difficult to deliver medications to ischemic areas in the brain.31 It is characterized by the hemorrhage or obstruction of cerebral blood vessels due to a lack of blood flow in the brain, leading to neurological impairment, oxidative stress, and brain cell malfunction.32 A series of degenerative processes is initiated by cerebral ischemia, leading to the eventual loss of neurons. Reperfusion is a therapeutic technique used to treat ischemic stroke and involves the infusion of tissue plasminogen activator (t-PA) or mechanical thrombectomy (MT).33 To overcome this problem, numerous neuroprotectants have been developed to reduce reperfusion-induced damage. However, all of them lack clinical approval because drugs cannot be effectively delivered to the brain through the BBB, drugs that circulate quickly, are unstable or poisonous, and it is difficult to select the proper drug regimen and dosages because of heterogenicity.31 Drug-loaded liposome-based NC systems, polymeric and artificial carriers, and inorganic NPs are formulations that are effective against ischemic stroke.
Brain Cancer
Brain cancer is the most fatal and invasive CNS disease worldwide. It is associated with various primary and metastatic CNS malignancies. Brain cancer is considered alarming, and its high recurrence rates and frequent resistance to therapy are painful signs of neurological degradation, resulting in lower survival rates.12 Glioblastoma multiforme (GBM), also known as grade IV malignant glioma, is an extremely invasive and aggressive tumor.34 Current treatment options for GBM include surgical resection, chemotherapy, and radiation. Owing to the ineffective therapeutic choices for GBMS, developing new methods and enhancing existing technology is crucial. Understanding the interactions between immune cells and malignant glioma cells, as well as the immunosuppressive properties of the tumor, is essential for a better understanding of the present difficulties. This interaction is governed by many variables that may be categorized as influencing factors for the BBB, immunosuppressive microenvironment, heterogeneity, subtype-specific glioblastoma genetic signatures, and irrelevant elements involved in modifying the impact of prior systemic therapy.35 Liposome NC and drug substances conjugated with inorganic NPs, such as dendrimer-loaded NPs are ideal nanomedicine materials for GBM therapy.1
How Advanced is Nanotechnology in Treating Brain Disorders?
Nanotechnology aims to design, create, and utilize nanomaterials with at least one dimension between 1 and 100 nm is the goal of nanotechnology.36 Superparamagnetic and surface plasmon resonances are two examples of bulk-independent phenomena typically present at this size and are of interest to the medical community.22 Interaction of proteins and nucleic acids with biomolecules and cells. They are similar in size to nanomaterials, particularly NPs.37 A high surface-to-volume ratio of the nanometric size enhances biological recognition. This improves the sensing, making detection more efficient and accurate. Recently, the use of nanomaterials in precision medicine has also increased38 (Figure 2 and Table 1).
![]() |
Figure 2 Diverse nano-based therapies for advancing brain disorder treatment. |
![]() |
Table 1 Current State of Nanomedicine in Treating Different Brain Disorders |
Nanocarrier for Drug Delivery
A pharmaceutical technique that has been developed to overcome these challenges uses virus-free NCs to deliver genetic treatments for brain disorders.48 Inorganic NPs, including gold and iron oxide NPs, polymer-based NPs, micro/nanoemulsions, scaffolds, drug-loaded nanoemulsions, dendrimers, and carbon nanotubes, have been proposed and studied as NCs (Figure 2 and Table 1).1,49 Nanomedicine-based gene delivery methods have several advantages over viral vectors, including improved targeting specificity, longer blood circulation, high loading capacity, manageable drug release, and decreased immunogenicity.50 The sole treatment for brain disorders is symptomatic relief because the medication cannot overcome the BBB.51 Nanotechnology-based therapy can overcome this limitation because of its advantages.52 Several different types of NCs have received approval from the FDA to be used with commercially available drugs.
Liposome
Spherical liposomes include a phospholipid bilayer and a water-soluble 100–400 nm core. By increasing lipophilicity, this bilayer helps macromolecules cross the blood-brain barrier.53 Liposomal NPs offer several therapeutic advantages. NPs are commercially available and are easily accessible to researchers and clinicians. Additionally, the synthesis and encapsulation of therapeutic compounds within liposomal NPs is relatively simple, allowing for efficient production. One notable advantage of liposomal NPs is their enhanced solubility as therapeutic agents. Passive diffusion can be initiated once the liposomes enter the brain. Passive efflux initiates this process.54 Apolipoprotein E (ApoE2) is delivered to the AD-damaged brain using a liposome carrier system modified with a mannose ligand and cell-penetrating peptides. The results showed that functionalized liposomes could safely and effectively transfer high gene concentrations to target tissues to treat AD.55
Polymeric Nanoparticles
The polymeric NPs had a size range of 1–100 nm. Coupling of therapeutically active compounds to enable targeted drug administration for the treatment of brain gliomas has been extensively investigated in research studies.56 Intratumoral delivery of anticancer drugs using NPs can treat malignant brain gliomas, such as glioblastoma multiforme.57 In animals with GBM, Convection-enhanced delivery (CED) and NP encapsulation improved drug penetration, distribution, and survival.58 The intravenous injection of Dox into poly(butyl cyanoacrylate) NPs led to increased drug accumulation at glioma rat tumor site.59 The most innovative method of drug delivery, particularly for NDs, is the use of polymeric NPs. These block-copolymeric compounds are easily eliminated without posing a systemic toxicity risk because they comprise monomers.60 These polymers are ideal for NP synthesis because of their biocompatibility and biodegradability.61 Recent research has focused on using curcumin-loaded PLGA NPs to improve drug delivery and reduce inflammation and oxidative stress in the treatment of AD.62 Polymeric biodegradable NPs functionalized with an antibody and polyethylene glycol (and) in transgenic AD mice.63 Exposure to PEGylated NPs can improve memory impairment and considerably reduce A-soluble peptide levels.64
Dendrimers
Dendrimers, which are nanosized and highly specific formulations, may cure neurodegenerative diseases.65 The size, core-shell composition, and surface functional groups of dendrimers can be altered to construct brain-delivered medication and gene NCs.66 The anti-amyloidogenic properties of the dendrimers may help treat prions, PD, and AD. Owing to their properties, dendrimers are medical equipment that sterilize solutions. Phosphorus-containing dendrimers have also been explored for the prevention of prion infection prevention.67 Misfolded prions can cause various brain diseases, making healthcare difficult. Many benefits arise from dendrimers.68 Dendrimers are highly branched nanoscale molecules with well-defined structures that are used for precise property control. This structural regulation allows the addition of phosphorus, which prevents prion infections.69 Dendrimers may assist in the systemic administration of AD medicines. Recent research suggests that many factors limit the production of this product, notably its high production costs. Further research is required to understand the health effects of extended dendrimer exposure.70
Micro/Nanoemulsions
Micro/nanoemulsions with 10–100 nm hydrophobic core-and-hydrophilic block copolymer shell structures can control anticancer drug release.71 This unique shell design prolongs drug circulation and prevents drug-conjugated core-phagocytosis-complement cascade interactions.72 Shells are ideal for PEG because they do not interact with serum proteins.73 The lack of targeting moieties hinders polymeric micelle-based GBM treatment, which may enhance tumor formation.67 Drinking water containing micelle-water-soluble coenzyme Q10 (Ubisol-Q10) in double transgenic AD mice. It improves long-term memory and lowers A plaques. Micro/nanoformulation helps target cells break down tau protein and activate autophagy.22 Curcumin-loaded polymeric nanoformulations and bovine serum albumin glycation in phosphate-buffered saline reduce AD-mice amyloidogenesis.74
Gold and Silica Nanoparticles
Nanomedicine has focused on Au NPs containing gold cores because of their advantages. These characteristics, including a high surface-to-volume ratio, blood-brain barrier penetration, and adjustable size, enable simple customization. AuNPs are among the most effective inorganic metal NCs for treating high-grade gliomas (HGG). The potential for treating HGG using AuNPs is limited because of their inability to target tumors.75 Through the recent trapping of Au NPs via gold-sulfur interactions, the ability of a DNA aptamer to target the expression of EGFRvIII in GBMs has been enhanced, and this new complex has been demonstrated as a unique possibility for GBM therapy in vivo and in vitro.76 AuNPs facilitate the delivery of targeted therapeutic genes.77 For instance, it showed better sensitization of GMB cells in mice using a new Au-iron oxide NP to deliver therapeutic miR-100 when administered with systemic TMZ.57 Clinical trials have been conducted for the treatment of HGG using a variety of AuNPs and chemotherapeutic drugs have been conducted.77
Drug-Loaded Nanoemulsion
Recently, there has been a significant surge in interest in the chemical and biological properties of inorganic NPs falling within the size range of 10–1000 nm.78 Drug delivery is promising because of the unique properties of NPs. These include their remarkable drug-carrying capacity, stability, controlled drug release, high selectivity in targeting cells or tissues, and the ability to transport hydrophilic and hydrophobic compounds. These advantages solve various problems associated with conventional drug delivery.79 The release of drug-loaded NPs into the target region may occur through diffusion, degradation, erosion, or external energy input.80 Targeted medication delivery frequently involves the use of ceramic NPs and proteins.38 The two most important criteria to consider when choosing an efficient method to create varied-sized NPs are the easy-to-functionalize property and strong biocompatibility of the changed molecule.81 The use of AuNPs provides a flexible platform for efficient drug delivery. By overcoming multidrug resistance (MDR), doxorubicin-coated AuNPs have shown improved drug accumulation in cancer treatment.82
Carbon Nanotube (CNT)
At a nanoscale size of less than 100 nm, CNT exhibits advanced physical, mechanical, and high-aspect-ratio characteristics.83 These factors significantly affect the cellular internalization of therapeutic compounds. Strategies for CNT functionalization include linking the carboxyl group after oxidation and adding an organic group to the sidewall or tip of the CNT.84 Additionally, CNTs linked to polymers and dendrimers exhibit improved biocompatibility, increased solubility, and decreased aggregation.85 Although acetylcholine-loaded SWCNT (single wall carbon nanotubes (SWCNTs) have been investigated for AD treatment, and CNT with stem cell therapy have been employed in treating stroke, only a few investigations of CNT in CNS treatment have been documented.86 The application of nanotechnology in biosciences and pharmaceutical businesses has been enhanced by modifying carbon nanohorns and nanodiamonds (CNT).87
How Do Recently Developed Methods Target Brain Disorders?
Scaffolds
Scaffolds of self-assembled peptide nanofibers have been investigated to enhance optic nerve regeneration.82 Nanofiber scaffolds were naturally produced by the peptides at salinity concentrations.83 Simple L-amino acids confer biocompatibility to these scaffolds.84 To evaluate its applicability, in vitro brain neuron synapse formation and PC12 cell neurite outgrowth were supported by a nanofiber scaffold composed of Arg–Ala–Asp–Ala (RADA) peptides.85,86 The quantity A scaffold, based on RADA (arginine-alanine-aspartic acid-alanine) enhanced axon regeneration across the lesion site in a hamster model of optic tract transection.65,87 This demonstrated that the capacity of the optic nerve to regenerate persists even after damage. Notably, this function was not enhanced by nanoscaffold therapy. The absence of intact ganglion cell axons in the transaction scenario or neuronal injury from the Mn2+ contrast agent are the two potential explanations proposed by the authors for the observed result.88 However, optimizing nanofiber technology may help the CNS heal after injury, possibly in combination with other techniques that provide biological signals to regenerated axons.89 The potential of the longitudinally structured nanoscaffolds for axonal regeneration was investigated. In one study, a nanofiber scaffold composed of gelatin and crosslinked dextran sulfate was employed.85 This scaffold was infused with human embryonic spinal cord cells, which enhanced the functional recovery in a rat spinal cord injury (SCI) model, including transection.90 The reinforcement of the scaffold was also observed. Self-assembling nanofiber scaffolds are another topic of study in SCI.91 Neural stem cell (NSC)-seeded scaffolds exhibit greater axonal innervation than nonseeded scaffolds. Animal implants using Schwann cell-seeded scaffolds showed significantly improved innervation compared with NSC scaffolds.92
3D Printing
Improvements in 3D printing technology and the creation of custom biomaterials have enabled the precise fabrication of biological components and intricate 3D geometrics precisely.88 CNS is vulnerable to several types of harm, including neurodegenerative disorders such as AD, PD, stroke, accident-related injuries, and brain cancer.89 With 3D printing, the peripheral nervous system (PNS) and nerve guidance conduits (NGCs) may be efficiently repaired, minimizing scarring and nerve compression, while serving as a bridge to replace larger gaps.90 A Fickian diffusion process causes the release of levodopa from the 3D-printed scaffolds over 14 days through MTT analysis, fluorescence microscopy, and SEM tests; mesenchymal stem cells (hAD-MSCs) generated from human adipose tissue were employed, and these techniques successfully verified appropriate biocompatibility. Overall, the results demonstrate that neural tissue engineering applications can employ PLA/CS 3D-printed scaffolds as a substitute for the levodopa delivery method for PD.91
4D Printing
Current printing technologies struggle to achieve nanoscale 3D printing. Nanocomposites, host material matrices with NPs, are the focus of 3D printing nanomedicine research. 3D printing has evolved owing to rapid advances in material science and additive manufacturing. Therefore, 4D printing has improved significantly. Time, which is the fourth dimension, allows 3D-printed objects to be distorted after printing. This fast-growing technology has produced dynamic and adaptable products that respond to external inputs. The applications and effects of AM are being studied.92 4D printing can be performed in two ways. One possibility is to include the transformation of information into the structural design. Using this technique, heterogeneous ink components are precisely positioned within multiple layers, enabling printed objects to self-morph.93 Alginate hydrogels allow for the effective bioprinting of Schwann cells, which express neurotrophic factors and help with peripheral nerve regeneration.94 Nanomaterials’ unique physical and chemical properties of nanomaterials have drawn attention to their potential to advance 4D printing.95 Nanomaterials can change the shear thinning of bioinks, thereby enabling 4D printing. The medical applications of 4D printing, particularly in tissue regeneration, are noteworthy. Scaffold manufacturing for this purpose must be explored further. Tissue regeneration involves spontaneous changes that can affect functional recovery.95 3D printing can create tissue scaffolds with complex geometries and microarchitectures; however, natural tissue regeneration mechanisms cannot be replicated. Stimuli-responsive 3D tissue constructs were produced using 4D printing. This discovery potentially replicates the tissue regeneration dynamics. Owing to cell traction stress, 4D metamorphosis may affect cell shape, orientation, density, and cell-to-cell communication. Therefore, tissue regeneration may benefit from these actions.96
5D and 6D Printing
The terms “5D” and “6D” printing refer to theoretical expansions of 3D printing that include the addition of dimensions to the printing process beyond the standard three spatial dimensions (x, y, and z). These technologies have the potential to markedly change the design and creation of complex structures, including biological tissues; however, they are still in the research stage and have not yet been extensively implemented. Precisely controlling printing parameters such as temperature, pressure, and speed is crucial to ensure optimal cell viability and functionality in 5D printing.97 Time and material properties are additional dimensions in 5D printing, meaning that the printed object can change over time or in response to different stimuli such as temperature, humidity, or light.98 The focus is on leveraging the more proven 4D and 5D printing processes to make biomaterials, although the idea of 6D printing is relatively new. Theoretically, combining 4D and 5D techniques in a coordinated manner can result in 6D printing. However, practical implementation is currently not feasible owing to insufficient knowledge and production capacity. Finding or creating the best material that is both stiff and responsive to one or more stimuli is the biggest challenge. The creation of structures with extra dimensions, such as topology and texture, is made possible by 6D printing, which can improve the mechanical, biological, and physical qualities of printed tissues.99
How Do Nanotechnology-Based Therapeutic Approaches Target Brain Disorders?
Nano-Immunotherapy
Therapeutic approaches targeting the immune system have achieved significant clinical success. Through the active detection and eradication of malignant cells, the immune system can inhibit.100 Nano-immunotherapy has become a viable approach to maximize the uptake of therapeutic drugs in brain disorders and decrease off-target damage, nano-immunotherapy has become a viable approach.101 Nanomaterials can assist in changing how biologics interact with target immune cells, resulting in the accumulation of these immunomodulators in brain disorders and the surrounding microenvironment.12 Nanomaterials can also target immunomodulators in brain disorders.
Nano Gene Therapy
Gene therapy has recently gained attention as a potential treatment for brain disorders caused by genetic mutations (Figure 3).12,102 Small interfering ribonucleic acids (siRNAs) and micro-ribonucleic acids (mRNA) can be used as therapeutic agents to modify gene expression with high specificity. For example, siRNAs can increase the susceptibility of cells to low molecular weights.103 A standard intracerebral injection may result in neurological injury and inadequate transgene expression due to the BBB.104 Several delivery methods have been reported for glioblastoma multi-forme gene therapy (Figure 3).105 A non-viral gene delivery strategy targeting angiogenesis has been established for effective gene therapy without harming healthy brain regions.106 Temporary gene transfer was accomplished using cationic microbubbles that were VEGFR2-targeted and exposed to focused transcranial ultrasound. Cationic microbubbles have been advertised for their high affinity and efficiency in loading DNA into cells of brain disorders in vitro.104 Recent advances in nano carrier-based gene therapy have enabled scientific organizations the capability and motivation to assess a wide range of prospective therapeutic options (Figure 3).107 In this context, nanomaterials as gene carriers have significantly increased the efficacy of gene therapy and minimized the enzymatic degradation of genetic components.108 They initially researched in vivo gene delivery to brain disorders using organically modified silica NPs (ORMOSIL) (Table 2).109 Numerous studies are being conducted to develop gene therapy approaches for treating neurological illnesses. Figure 3 shows that gene therapies have to cross the BBB to be effective. The endothelial membrane forms a biological barrier in the brain vasculature. To prevent the passage of chemical substances, such as toxins and harmful microbes, endothelial cells are joined together by tight junctions.110,111
![]() |
Table 2 Multiple Routes of Nano Pharmaceuticals in Treating Brain disorders |
Interdisciplinary integration of efforts to build nanotechnology will help elucidate the function of neural circuits and devise methods for diagnosing and treating brain illnesses.119 In AD, encapsulated synthetic NPs of poly(lactide-co-glycolic acid) is used as an effective agent. Similarly, in vitro studies have shown that polymeric NPs increase drug delivery to the brain and reduce oxidative stress, inflammation, and plaque burden.120 Poly(ethylene imine)-based NPs are used for delivering genes for gene therapy in brain cancer, including poly(ethylene imine)-poly(L-lysine) as a copolymer reported as a gene delivery systems, and in vitro studies have shown that the incorporation of L-glutathione acts as a backbone for poly(ethylene imine), which increases cytocompatibility and improves the ability of the polymer to cross the BBB.121,122 Additionally, liposomes are considered carriers for gene therapy. Liposomes and mannitol act as delivery systems to transfer oligonucleotides for treatments that would cross the BBB in brain cancer.
Similarly, a PEGylated dendrigraft poly-L-lysine was used to develop a multifunctional NC that could carry desired peptides and genes to the brain via systemic injection (Table 2).123 Solid lipid NPs and coated and non-coated chitosan were used to transfer siRNA from the nose to the brain via the trigeminal and olfactory nerve routes. A Caco-2 epithelial cell model was used to release siRNA, which successfully entered the monolayer to target BACE1.124 In another study, PEGylated magnetic NPs were utilized to deliver the siRNA to nerve cells by endocytosis to suppress the BACE1 gene and reduce Aβ production.54 They examined the viability of using cutting-edge technology to compress DNA plasmids into NPs and send them to the brain to slow or stop neurodegeneration in an in vivo model of PD.125
Similarly, α-synuclein is embedded in multifunctional magnetic NPs containing a small hairpin RNA, and it efficiently repairs PD.126 Gene therapy is beneficial owing to its ability to deliver gene therapy medications to target brain areas that can alter functions and cure PD without causing side effects.127 CRISPR-Cas9 was used to knock out Vps35 in exon 15 of the mouse PARK17. This has a direct impact on easing PD symptoms by considerably reducing the release of dopamine from the substantia nigra in the middle of the brain.128 Superparamagnetic iron oxide NPs (SPIONs) are used as nanovehicles for cell tracing and gene therapy in stroke.
How Do BBB Crossing Strategies Use Nanomaterials?
NPs are advantageous in medical delivery owing to their numerous advantages, including extended bloodstream circulation, regulated medication release, enhanced stability, high drug-loading capacity, and targeted delivery (Figure 3). Consequently, the potential of nanomaterials for transferring therapeutic medications across the BBB has been thoroughly investigated.129 The size, zeta potential, and hydrophilicity of nanomaterials have a major impact on their in vivo.130,131 Nanomaterial-based BBB crossing mechanisms and strategies, BBB transportability study techniques, and treatment for NDs, such as AD and PD, frequently fail because chemicals have trouble entering the brain due to the BBB.132 Numerous drug delivery techniques demonstrate the successful transportation of diverse pharmaceutical agents across the BBB using nanomaterials.133 This study used nanomaterials to demonstrate and analyze ways to bridge the BBB.
Intranasal Delivery
The intranasal route of drug delivery is a productive and non-invasive method for delivering medications to the brain, circumventing the challenges associated with parenteral administration.134 By employing an intranasal administration method, drugs can be efficiently transferred to the brain through the olfactory mucosa.135 This can occur by traversing the connective tissue surrounding the olfactory nerve bundle or the axons of olfactory neurons, thereby circumventing the BBB.136 Intranasal administration has successfully circumvented hepatic first-pass metabolism, reduced drug accumulation in non-target tissues, and mitigated systemic side effects.137 Furthermore, intranasal administration has emerged as a widely utilized approach in pharmaceutical delivery owing to several benefits, including prompt absorption, rapid initiation of action, noninvasive nature, minimal tissue damage, and straightforward application.138 This study revealed significant variations in the effectiveness of drug delivery among different intranasal delivery nanomaterials. These variations were mostly independent of the physicochemical features of the pharmaceuticals delivered.139
Temporary Disruption of the BBB
Temporary rupture of the BBB has been extensively investigated as a prominent strategy for facilitating the delivery of medications from the circulatory system into brain disorder.140 The methods used to disrupt the BBB mostly involve osmotic, ultrasonic, and magnetic disruption.132
Local Delivery
Numerous studies have been conducted on the direct administration of medications for local brain disorders. Numerous procedures involving localized delivery of various therapeutic drugs have been established. Early research on local non-drug delivery into the CNS mostly used the implantation of biodegradable polymer wafers with a pharmacological payload.141 These wafers are designed to release drugs in a controlled manner over an extended period, leading to enhanced therapeutic outcomes.
Cell-Penetrating Peptide-Mediated Blood-Brain Barrier Crossing
Cationic cell-penetrating peptides (CPPs) are short and amphipathic and have cationic properties.142 Several peptides have been shown to increase the translocation of conjugated drugs or biomaterials through cell membranes.143 There are two primary categories of cationic CPPs: Antimicrobial sequences and chimeric peptides.144 Although the precise mechanism by which CPPs facilitate cell membrane transportation remains unclear, it is believed that electrostatic interactions between the cationic charges present on CPPs and the surfaces of brain endothelial cell membranes play a crucial role in enabling the transport of CPP-modified nanomaterials across the BBB.145
Receptor-Mediated BBB Crossing
The receptor-mediated transcytosis pathway transports numerous endogenous macromolecules into the brain.146 The interactions between ligands and their corresponding receptors on the surface of brain endothelial cells (BECs) facilitate vesicle formation through endocytosis pathway.147 Subsequently, the vesicles release ligands, allowing them to cross the BBB and enter the CNS via exocytosis. This allows the ligands to perform their biological roles in the CNS.103
Shuttle Peptide-Mediated BBB Crossing
There is accumulating interest in shuttle peptides owing to their cost-effectiveness, abundant availability, diminished immunogenicity, and notable chemical adaptability, such as their compatibility with chemical alterations.10 Shuttle peptides facilitate the translocation of diverse payloads across the BBB, encompassing tiny molecules, genetic material, proteins, and NPs.148 Numerous BBB shuttle peptides have been devised to facilitate the transport of NPs, thereby enhancing their dispersion within the CNS.
BBB Crossing Mechanism
The BBB, blood–CSF barrier (BCB), and arachnoid barrier are the three major interfaces formed by distinct cells. Microvascular endothelial cells that line the cerebral capillaries and enter most mammalian brains, spinal cords, and other organisms with a fully developed CNS constitute the BBB. Given that the average adult total surface area falls between 12 and 18 m2 and the average microvessel surface area per gram of tissue is between 150 and 200 cm, it is regarded as the largest interface for blood–brain exchange. BBB is essential for shielding the brain parenchyma from blood-borne pathogens and acts as a formidable barrier against the entry of medications and other exogenous substances into the CNS.149
Three methods—non-invasive, invasive, and identifying alternate drug delivery pathways—can transport a nanomaterial-based BBB throughout the brain.150 Noninvasive methods widely used in research depend on biological processes to help drugs pass through transcellular channels and the blood-brain barrier (Figure 3). 151 The intranasal delivery method effectively crosses the BBB by delivering NPs through the olfactory epithelium or trigeminal nerves directly into the BBB.152 However, this is challenging for large proteins to do so. With low cellular toxicity and high effectiveness, cell-penetrating peptide (CPP)-based delivery methods can successfully transport macromolecules across cellular membranes.138 The BBB must be physically broken apart for the invasive mechanism to function, and nanomaterials must be transferred along paracellular channels to cross the BBB (Figure 3).153 The transient BBB disruption technique described above and local delivery strategy are part of the invasive mechanism, also known as the paracellular mechanism.154 In the non-invasive approach, the BBB is unaltered during the drug delivery process, and NPs are delivered across the BBB via transcellular channel.155 Noninvasive or transcellular intranasal delivery strategies include four BBB crossing methods: CPP-mediated, receptor-mediated, shuttle peptide-mediated, and cell-mediated.
In contrast, invasive procedures manipulate the BBB or directly administer medications to the brain tissue.156 Various methods have been used in invasive research, including intracerebral implants and intraventricular, intrathecal, and interstitial deliveries. Researchers have targeted medications for convection-enhanced delivery. The use of osmotic methods to cross the BBB is invasive. Pharmacological and ultrasound-mediated BBBD have also been used in invasive research.1 S-mediated BBBD uses hyperosmotic solutions, concentrated ultrasound, and magnetic fields to shrink endothelial cells and destroy tight junctions, allowing molecules to cross the BBB.157
Nano Protein/Enzyme Replacement Therapy
NPs can incorporate moieties into a single therapeutic molecule, making them potential treatments for brain diseases. This method delivers multiple medicinal molecules simultaneously, improving the treatment efficacy.158 The chemical and biological features of NPs make them the most versatile delivery options. NPs comprise lipids, polymers, dendrimers, and inorganic compounds. The core material used to finely regulate the NP size, shape, charge, binding capacity, and hydrophobicity/hydrophilicity (internally and externally) is crucial.125 The relationship between these parameters and the pharmacokinetic half-life, biodistribution, stability, and binding capability of therapeutic proteins or enzymes has been thoroughly examined (Figure 4).14,159 When coupled with seq-containing proteins, the cationic tail promoted NP production.8 The potential use of these particles with many therapeutic proteins is crucial for their study and development. However, healthy mouse models have shown that targeted NPs accumulate in the brain, similar to non-targeted NPs.160 The study reported uptake into proinflammatory Th17 cells with no harmful effects at all doses. Compared to nanospheres alone, nanospheres reduced interleukins and improved clinical scores (Table 3).161 However, these studies did not include long-term observations. Nevertheless, nanospheres affect different body regions, triggering a brain reaction to autoimmune disorders.162
![]() |
Table 3 Some Brain Disorder Drug Delivery Features of Nanoparticles with Patents |
Nasal medication delivery has been proposed to improve the delivery of protein-loaded NP brain delivery. This procedure is less intrusive than a direct injection.168,169 This approach must meet certain criteria to be deemed viable. Nasal administration, such as direct injection, bypasses first-pass clearance and allows therapeutic drug distribution to the CNS via the olfactory bulb and brainstem.170 The co-administration of matrix metalloproteinase-9 (MMP-9), a chemical that increases nasal epithelial permeability, increases the efficiency of brain medication delivery. The therapeutic chemical was delivered twice to the midbrain and cortex, and three times to the brainstem. However, nasal delivery systems have several disadvantages. Previous studies have found that the administration of nasal medication is hindered by nasal epithelial absorption and brain tissue transfer (Figure 4). The improved delivery strategy reduced stroke infarct size by 50% and increased life expectancy by 21 days compared to intravenous and intranasal exosome administration (Table 3).171 Fluorescence imaging showed that intranasal administration resulted in a much higher absorption rate than intravenous administration. Active microglial cells were reduced to levels comparable to control animals.172 However, therapeutic applications of these improvements are limited. Thus, the treatment of brain disease must be improved.173 The physical properties of NPs make them suitable for encapsulating enzymes. The stability, cellular uptake, and activity of enzyme-loaded NPs have been extensively explored.174,175
However, the development of enzyme-loaded NPs for brain administration has been limited compared liposomes, PBCA, and PLGA NPs.168 anti-NR1-SODPBCA NPs were targeted using an antiNMDA receptor 1 antibody. In this study, the antibody levels varied significantly between the vehicle types. Notably, all particles accumulated in the brain. This caused a 50% reduction in infarct size in the mouse ischemia model. GNeo-NHS-modified 1,2-dioleoyl-snglycero-3-phosphoethanolamine (DOPE)176 was encapsulated with positive and negative charges in another liposome. Fibroblasts were treated with L-iduronidase to examine the effects of glycosaminoglycan. Effective targeted strategies to help these NPs breach the BBB are needed to advance in vivo investigations.177,178
How is One Possible Therapeutic Approach Individualized Through Nanomedicine?
Tailored nanomedicines have gained considerable scientific interest in recent years owing to their considerable potential for safe, precise, and regulated drug administration across the BBB, tailored nanomedicines have gained much scientific interest in recent years.85 Tailored nanomedicines may offer many advantages in treating brain disorders and neuro-AIDS problems.179 In addition to on-demand drug release, personalized medicine is appealing because it allows precise adjustment of the therapeutic dose for each patient.180 Additionally, the incorporation of longitudinal monitoring techniques can support the tracking of patient responses to therapeutic interventions, drug doses, and treatment protocols, enabling doctors to customize their treatment plans.181 Personalized nanomedicines offer significant potential to achieve maximal efficacy with the least side effects, regardless of whether they are used to treat brain disorders.182 The development of stimuli-responsive nanomedicines, which include biological, chemical, physical, dual, and multi-responsive forms, has enabled site-specific accumulation and triggered drug release.183
Furthermore, studies on the application of prodrug-based nanomedicines have provided several benefits, including better drug loading efficiency, increased targeting capacity, real-time control, and reduced risk of side effects.132 Several events can activate nanomedicines. Nanomedicine steric shields built of stimuli-responsive cleavable polymers can prolong tumor circulation and effectively administer medication to target cells. Table 3 shows that patents approved by the FDA and NIH have created a customized medicine roadmap to transform healthcare. Personalizing patient treatments and interventions is the goal of this research to change healthcare. These companies use genomes, technology, and data analyses to improve healthcare accuracy, targeting, and patient centricity. These esteemed university partnerships show that personalized medicine is a promising way to satisfy healthcare needs and improve health outcomes.184
Aspects of Nanomaterials and Nano Therapies’ Biosafety
The unique physicochemical features of nanomaterials can improve brain cancer treatment.12 Nano-pollution results show that NPs are easily absorbed by cells owing to their small size. Soft NPs made of polymers and liposomes have gained regulatory permission for clinical use and have demonstrated promise in nanomedicine.185 In contrast to their use as therapeutic agents, NPs are mostly studied as delivery agents, and the effects of NPs on biological systems after administration and their removal still need to be thoroughly studied.186 Before NPs are used in clinical settings, it is important to study several preclinical and clinical trial design problems such as immediate and long-term stability, tolerability, biodistribution, and elimination of NPs.187 Publications on clinical trials of nanomedicine that are currently available generally concentrate on the therapeutic effectiveness of pharmaceuticals, ignoring the potential negative consequences of NPs on human health.188 The NanoTherm® therapy was developed by a German company named MagForce. It employs magnetic hyperthermia and radiation to destroy cancer cells using a ferrofluid consisting of superparamagnetic iron oxide NPs.72 Nanotherms provided an average survival of 13.2 months in a clinical trial involving 60 patients, compared to 6 months with standard care.189. As the FDA has not provided any guidelines for particular nano-based products, regulatory authorization of nano-therapeutics presents additional, significant obstacles.190 Currently, the conditions used have been steadily established under regulations on bulk commodities. The monitoring results for nanoformulated medications are based on a discrete assessment of benefits and risks, which creates a difficult position and delays commercialization.12
However, the lack of regulatory rules and standards for engineered nanomaterials is a crucial issue that must be addressed.191 National/international agencies must make thoughtful decisions and forward them to create recommendations that can help researchers investigate potential medicinal applications. Additionally, it is crucial to create interdisciplinary partnerships based on public and private experts to advance the translational elements of nanomedicines while considering the clinical environment.192 Notably, as already mentioned, strong partnerships and the standardization of nanomedicine as a form of treatment entail investigating the physiological aspects of drug NCs, the effects of differential stimulation of biological functions, increased efficacy, absence of adverse effects, and knowledge of the benefits of nanomedicine.193 These factors will be crucial in bridging research gaps and facilitating successful translation from laboratory to clinical settings.
Challenges and Alternative Approaches
The development of multifunctional nanomaterials with specific and tunable features for nanotherapeutics has created substantial obstacles that require immediate attention.12 Building on decades of progress, nanotherapies for brain disorders are predicted to alter healthcare systems. The methodologies and achievements of nominal cancer nanotherapeutics have limitations and problems.194 Several nanoformulations have been used in clinical studies. Their efficiency, biocompatibility, minimal side effects, and low toxicity distinguish these formulations.195 Biochemical classification includes organic and inorganic NCs is currently being tested in preclinical trials to improve survival and decrease tumor growth.196 NanoTherm®, developed by German business Magforce, has advanced cancer treatment. This novel treatment uses magnetic hyperthermia and radiotherapy to target and kill cancer cells using superparamagnetic iron oxide NPs, specifically ferrofluid.197 In a clinical trial of 60 patients, nanotherms improved survival by 13.2 months compared with 6 months with conventional treatment.136 The National Library of Medicine ClinicalTrials. gov database lists 2236 brain cancer. Two-hundred thirty-eight of these studies used nanotechnology in their study. A preliminary clinical trial of 10 individuals tested 89Zr-cRGDY ultrasmall silica particles. This study aimed to determine whether these particles could track brain disorder.198 Nanomaterial fabrication and characterization must be carefully considered when building drug delivery systems199 to reduce NC toxicity in healthy cells. This organized and defined process evaluates the advantages and disadvantages of these medications. Due to its intricacy, this evaluation can take time, delaying drug commercialization. The widespread use of multifunctional nanoplatforms is expected to exacerbate technological challenges.
Future Prospectives
Drug-loaded NC spose many challenges to researchers and deregulatory organizations. Robust characterization techniques, including optimization of methodologies, safety regulations, and stability upkeep, are required to address these issues.200 Recently, artificial intelligence (AI) has helped to improve NCs for neurological illnesses, which will help focus this issue known as “computational pharmaceutics”, created due to the exponential rise in computer power and algorithms over the previous ten years. Recently, AI and multiscale modeling has been used, suggesting a potential shift to drug delivery systems. NCs are advantageous for directing medications to certain cells or tissues, preventing toxicity, and triggering healthy cells.201 The size, shape, chemical composition, and surface characteristics of NCs play a role in how well they carry drugs. However, developing ideal nanocarriers for drug delivery is difficult.202 AI-based computational methods can help optimize the drug loading, retention, and stability of NCs. The majority of the focus of molecular modeling studies for nanocarrier-based drug delivery systems has been (i) analyzing the development and structure of NCs, (ii) assessing the interactions and distribution of NCs, (iii) assessing the surface qualities of nanocarriers, and (iv) the adsorption of nanocarriers onto various surfaces.203
Furthermore, each NCs can be improved to exhibit the desired behavior. Thus, creating a repository that aids in identifying a suitable NCs scaffold and its functional groups for encapsulating and releasing a particular medication is a significant advancement. In the “Collaboratory for Structural Nanobiology”, efforts have been undertaken to establish a database repository of NCs, where scientists may access the 3D structures and physical and chemical characteristics of NCs.204 NanoAmpere is a comprehensive database that details the security.205 Although computational research and AI will never replace laboratory experiments, they play a crucial role in speeding up and improving target and drug discovery processes, as well as inventing new, powerful computational tools for DDS simulation and NPs, which are constantly evolving. Unique regulations are required for the industry because of the potential implications for the global economy and the necessity to provide safety evaluations for nanomedicine therapy in neurological disorders.
In conclusion, there is a need for standardized norms and controls for NPs. To address this concern, national and international organizations must make informed decisions and establish comprehensive criteria for the use of nanomaterials in medicinal applications. Researchers in this field will significantly benefit from these guidelines. Interdisciplinary collaboration between the public and private sectors is also necessary to translate nanomedicine into clinical practice, particularly in healthcare. Major research fields include the ongoing pursuit of nanomedicine therapy standardization and robust cooperation. These studies examined the physiological effects and biological activities of drug NCs to enhance their biological efficacy and safety. Raising awareness about the benefits of nanomedicine is crucial for translating research findings into clinical practice. This comprehensive study summarizes the significance of nanotechnology in various therapeutic approaches including immunotherapy, stem cell therapy, gene therapy, and tailored nanomedicine for brain disorders. This review emphasizes that the unique physicochemical features of NPs can overcome obstacles in drug-free therapies, such as photodynamic treatment (PDT) and radiation, address challenges such as radio-resistant treatment, and reduce mortality risks. However, it is imperative to consider the biosafety of nano-assisted medicines prior to clinical translation. Assessing factors such as biodistribution, pharmacokinetics, and toxicity is crucial for the safe use of these medicines. In summary, interdisciplinary collaboration, awareness, and biosafety considerations key to advancing nanomedicine toward practical clinical applications.
Acknowledgments
The authors acknowledge the Indian Council of Medical Research (ICMR) for the research grant support toward the project titled “Formulation and characterization of diabetic wound healing gauze using PDGF-eugenol nanocomposites for Diabetic foot ulcer (DFU) – A 3D bioprinting approach” (file no. EM/Dev/SG/144/2109/2023). Priyadarshini Mohapatra also immensely acknowledge ICMR, Government of India for Senior Research Fellowship [File No. 3/2/2/45/2020-NCD-lll] and Vellore Institute of Technology for giving the laboratory facilities to carry out the research work.
Author Contributions
All authors made a significant contribution to the work reported, whether that is in the conception, study design, execution, acquisition of data, analysis, and interpretation, or in all these areas; took part in drafting, revising, or critically reviewing the article; gave final approval of the version to be published; have agreed on the journal to which the article has been submitted; and agree to be accountable for all aspects of the work.
Disclosure
The authors declare no conflicts of interest in this work.
References
1. Nguyen TT, Dung Nguyen TT, Vo TK, et al. Nanotechnology-based drug delivery for central nervous system disorders. Biomed Pharmacother. 2021;143:112117. doi:10.1016/j.biopha.2021.112117
2. Mehndiratta MM, Aggarwal V. Neurological disorders in India: past, present, and next steps. Lancet Glob Health. 2021;9(8):e1043–e1044. doi:10.1016/S2214-109X(21)00214-X
3. Upadhya M, Jimmy N, Jaison JM, et al. Drug utilization evaluation of medications used in the management of neurological disorders. Global Health J. 2023. doi:10.1016/j.glohj.2023.07.006
4. Van Giau V, An SSA, Bagyinszky E, Kim S. Gene panels and primers for next generation sequencing studies on neurodegenerative disorders. Mol Cell Toxicol. 2015;11(2):89–143. doi:10.1007/s13273-015-0011-9
5. Re F, Gregori M, Masserini M. Nanotechnology for neurodegenerative disorders. Maturitas. 2012;73(1):45–51. doi:10.1016/j.maturitas.2011.12.015
6. Bioscience biotechnology research communications. Emerging applications of nanotechnology in neurological disorders: recent review. Available from: https://bbrc.in/emerging-applications-of-nanotechnology-in-neurological-disorders-recent-meta-review/.
7. Chhabra R, Tosi G, Grabrucker AM. Emerging use of nanotechnology in the treatment of neurological disorders. Curr Pharm Des. 2015;21(22):3111–3130. doi:10.2174/1381612821666150531164124
8. Parveen S, Misra R, Sahoo SK. Nanoparticles: a boon to drug delivery, therapeutics, diagnostics and imaging. Nanomed Nanotechnol Biol Med. 2012;8(2):147–166. doi:10.1016/j.nano.2011.05.016
9. Kabanov AV, Batrakova EV. New technologies for drug delivery across the blood brain barrier. Curr Pharm Des. 2004;10(12):1355–1363.
10. Luo M, Lee LKC, Peng B, Choi CHJ, Tong WY, Voelcker NH. Delivering the promise of gene therapy with nanomedicines in treating central nervous system diseases. Adv Sci 2022;9(26):2201740. doi:10.1002/advs.202201740
11. Mohapatra P, Chandrasekaran N. Wnt/β-catenin targeting in liver carcinoma through nanotechnology-based drug repurposing: a review. Biomed Pharmacother. 2022;155:113713. doi:10.1016/j.biopha.2022.113713
12. Nehra M, Uthappa UT, Kumar V, et al. Nanobiotechnology-assisted therapies to manage brain cancer in personalized manner. J Control Release. 2021;338:224–243. doi:10.1016/j.jconrel.2021.08.027
13. Liu Z, Jiang W, Nam J, Moon JJ, Kim BYS. Immunomodulating nanomedicine for cancer therapy. Nano Lett. 2018;18(11):6655–6659. doi:10.1021/acs.nanolett.8b02340
14. Safary A, Akbarzadeh Khiavi M, Mousavi R, Barar J, Rafi MA. Enzyme replacement therapies: what is the best option? Bioimpacts. 2018;8(3):153–157. doi:10.15171/bi.2018.17
15. Muro S. New biotechnological and nanomedicine strategies for treatment of lysosomal storage disorders. WIREs Nanomed Nanobiotechnol. 2010;2(2):189–204. doi:10.1002/wnan.73
16. Grosso AD, Galliani M, Angella L, et al. Brain-targeted enzyme-loaded nanoparticles: a breach through the blood-brain barrier for enzyme replacement therapy in Krabbe disease. Sci Adv. 2019. doi:10.1126/sciadv.aax7462
17. Sun C, Ding Y, Zhou L, et al. Noninvasive nanoparticle strategies for brain tumor targeting. Nanomed Nanotechnol Biol Med. 2017;13(8):2605–2621. doi:10.1016/j.nano.2017.07.009
18. Olivier JC. Drug transport to brain with targeted nanoparticles. NeuroRx. 2005;2(1):108–119. doi:10.1602/neurorx.2.1.108
19. Rizvi SAA, Saleh AM. Applications of nanoparticle systems in drug delivery technology. Saudi Pharm J. 2018;26(1):64–70. doi:10.1016/j.jsps.2017.10.012
20. Iqubal A, Iqubal MK, Khan A, Ali J, Baboota S, Haque SE. Gene therapy, A novel therapeutic tool for neurological disorders: current progress, challenges and future prospective. Curr Gene Ther. 2020;20(3):184–194. doi:10.2174/1566523220999200716111502
21. Sundarrajan S, Venkatesan A, Kumar SU, et al. Exome sequence analysis of rare frequency variants in Late-Onset Alzheimer Disease. Metab Brain Dis. 2023;38(6):2025–2036. doi:10.1007/s11011-023-01221-7
22. Cao Y, Zhang R. The application of nanotechnology in treatment of Alzheimer’s disease. Frontiers in Bioengineering and Biotechnology; 2022. Available from: https://www.frontiersin.org/articles/10.3389/fbioe.2022.1042986.
23. Sood S, Jain K, Gowthamarajan K. Intranasal therapeutic strategies for management of Alzheimer’s disease. J Drug Target. 2014;22(4):279–294. doi:10.3109/1061186X.2013.876644
24. Sabbagh MN. Alzheimer’s Disease drug development pipeline 2020. J Prev Alzheimers Dis. 2020;7(2):66–67. doi:10.14283/jpad.2020.12
25. Wilczewska AZ, Niemirowicz K, Markiewicz KH, Car H. Nanoparticles as drug delivery systems. Pharmacol Rep. 2012;64(5):1020–1037. doi:10.1016/S1734-1140(12)70901-5
26. Lamptey RNL, Chaulagain B, Trivedi R, Gothwal A, Layek B, Singh J. A review of the common neurodegenerative disorders: current therapeutic approaches and the potential role of nanotherapeutics. Int J Mol Sci. 2022;23(3):1851. doi:10.3390/ijms23031851
27. Spires-Jones TL, Attems J, Thal DR. Interactions of pathological proteins in neurodegenerative diseases. Acta Neuropathol. 2017;134(2):187–205. doi:10.1007/s00401-017-1709-7
28. Schulz-Schaeffer W. The synaptic pathology of α-synuclein aggregation in dementia with Lewy bodies, Parkinson’s disease and Parkinson’s disease dementia. Acta Neuropathol. 2010;120:131–143. doi:10.1007/s00401-010-0711-0
29. Mavridis IN, Meliou M, Pyrgelis ES, Agapiou E. Chapter 1 - Nanotechnology and Parkinson’s disease. In: Grumezescu AM, editor. Design of Nanostructures for Versatile Therapeutic Applications. William Andrew Publishing. 2018:1–29. doi:10.1016/B978-0-12-813667-6.00001-2
30. Ganesan P, Ko HM, Kim IS, Choi DK. Recent trends in the development of nanophytobioactive compounds and delivery systems for their possible role in reducing oxidative stress in Parkinson’s disease models. Int J Nanomed. 2015;10:6757–6772. doi:10.2147/IJN.S93918
31. Dong X, Gao J, Su Y, Wang Z. Nanomedicine for Ischemic Stroke. Int J Mol Sci. 2020;21(20):7600. doi:10.3390/ijms21207600
32. Fornage M Grand challenges in stroke genomics. Frontiers in Stroke; 2022. Available from: https://www.frontiersin.org/articles/10.3389/fstro.2022.984176.
33. Tsivgoulis G, Katsanos AH, Alexandrov AV. Reperfusion therapies of acute ischemic stroke: potentials and failures. Front Neurol. 2014;5:215. doi:10.3389/fneur.2014.00215
34. Ostrom QT, Cioffi G, Gittleman H, et al. CBTRUS statistical report: primary brain and other central nervous system tumors diagnosed in the United States in 2012–2016. Neuro-Oncology. 2019;21(Supplement_5):v1–v100. doi:10.1093/neuonc/noz150
35. Khaddour K, Johanns TM, Ansstas G. The Landscape of Novel therapeutics and challenges in glioblastoma multiforme: contemporary state and future directions. Pharmaceuticals. 2020;13(11):389. doi:10.3390/ph13110389
36. Ijaz I, Gilani E, Nazir A, Bukhari A. Detail review on chemical, physical and green synthesis, classification, characterizations and applications of nanoparticles. Green Chem Lett Rev. 2020;13(3):223–245. doi:10.1080/17518253.2020.1802517
37. Kelley SO, Mirkin CA, Walt DR, Ismagilov RF, Toner M, Sargent EH. Advancing the speed, sensitivity and accuracy of biomolecular detection using multi-length-scale engineering. Nature Nanotech. 2014;9(12):969–980. doi:10.1038/nnano.2014.261
38. Zhang TT, Li W, Meng G, Wang P, Liao W. Strategies for transporting nanoparticles across the blood–brain barrier. Biomater Sci 2016;4(2):219–229. doi:10.1039/C5BM00383K
39. Prabhakar P, Ahmed ABA, Chidambaram SB. the role of phloridzin and its possible potential therapeutic effect on Parkinson’s disease. Int J Nutr Pharmacol Neurol Dis. 2020;10(2):69. doi:10.4103/ijnpnd.ijnpnd_80_19
40. Varallyay CG, Nesbit E, Fu R, et al. High-resolution steady-state cerebral blood volume maps in patients with central nervous system neoplasms using ferumoxytol, a superparamagnetic iron oxide nanoparticle. J Cereb Blood Flow Metab. 2013;33(5):780–786. doi:10.1038/jcbfm.2013.36
41. Gambaryan PY, Kondrasheva IG, Severin ES, Guseva AA, Kamensky AA. Increasing the efficiency of parkinson’s disease treatment using a poly(lactic-co-glycolic acid) (PLGA) Based L-DOPA delivery system. Exp Neurobiol. 2014;23(3):246–252. doi:10.5607/en.2014.23.3.246
42. Feng Y, Panwar N, Tng DJH, Tjin SC, Wang K, Yong KT. The application of mesoporous silica nanoparticle family in cancer theranostics. Coord Chem Rev. 2016;319:86–109. doi:10.1016/j.ccr.2016.04.019
43. Lux F, Tran VL, Thomas E, et al. AGuIX® from bench to bedside—Transfer of an ultrasmall theranostic gadolinium-based nanoparticle to clinical medicine. BJR. 2019;92(1093):20180365. doi:10.1259/bjr.20180365
44. Gilert A, Machluf M. Nano to micro delivery systems: targeting angiogenesis in brain tumors. Vasc Cell. 2010;2(1):20. doi:10.1186/2040-2384-2-20
45. Lochhead JJ, Thorne RG. Intranasal delivery of biologics to the central nervous system. Adv Drug Delivery Rev. 2012;64(7):614–628. doi:10.1016/j.addr.2011.11.002
46. Cui Z, Lockman PR, Atwood CS, et al. Novel d-penicillamine carrying nanoparticles for metal chelation therapy in Alzheimer’s and other CNS diseases. Eur J Pharm Biopharm. 2005;59(2):263–272. doi:10.1016/j.ejpb.2004.07.009
47. Wilson B, Samanta MK, Santhi K, Kumar KPS, Paramakrishnan N, Suresh B. Poly(n-butylcyanoacrylate) nanoparticles coated with polysorbate 80 for the targeted delivery of rivastigmine into the brain to treat Alzheimer’s disease. Brain Res. 2008;1200:159–168. doi:10.1016/j.brainres.2008.01.039
48. Jayant RD, Sosa D, Kaushik A, et al. Current status of non-viral gene therapy for CNS disorders. Expert Opin Drug Delivery. 2016;13(10):1433–1445. doi:10.1080/17425247.2016.1188802
49. Wei QY, Xu YM, Lau ATY. Recent progress of nanocarrier-based therapy for solid malignancies. Cancers. 2020;12(10):2783. doi:10.3390/cancers12102783
50. Suk JS, Xu Q, Kim N, Hanes J, Ensign LM. PEGylation as a strategy for improving nanoparticle-based drug and gene delivery. Adv. Drug Delivery Rev. 2016;99:28–51. doi:10.1016/j.addr.2015.09.012
51. Chopade P, Chopade N, Zhao Z, Mitragotri S, Liao R, Chandran Suja V. Alzheimer’s and Parkinson’s disease therapies in the clinic. Bioeng Transl Med. 2023;8(1):e10367. doi:10.1002/btm2.10367
52. Mirón-Barroso S, Domènech EB, Trigueros S. Nanotechnology-based strategies to overcome current barriers in gene delivery. Int J Mol Sci. 2021;22(16):8537. doi:10.3390/ijms22168537
53. Farooque F, Wasi M, Mughees MM. Liposomes as drug delivery system: an updated review. J Drug Delivery Ther. 2021;11(5–S):149–158. doi:10.22270/jddt.v11i5-S.5063
54. Lopez-Barbosa N, Garcia JG, Cifuentes J, et al. Multifunctional magnetite nanoparticles to enable delivery of siRNA for the potential treatment of Alzheimer’s. Drug Deliv. 2020;27(1):864–875. doi:10.1080/10717544.2020.1775724
55. Arora S, Layek B, Singh J. Design and validation of liposomal ApoE2 gene delivery system to evade blood-brain barrier for effective treatment of Alzheimer’s disease. Mol Pharmaceut. 2020. doi:10.1021/acs.molpharmaceut.0c00461
56. Sahoo NG, Rana S, Cho JW, Li L, Chan SH. Polymer nanocomposites based on functionalized carbon nanotubes. Prog Polym Sci. 2010;35(7):837–867. doi:10.1016/j.progpolymsci.2010.03.002
57. Kurawattimath V, Wilson B, Geetha KM. Nanoparticle-based drug delivery across the blood-brain barrier for treating malignant brain glioma. OpenNano. 2023;10:100128. doi:10.1016/j.onano.2023.100128
58. Vogelbaum MA, Aghi MK. Convection-enhanced delivery for the treatment of glioblastoma. Neuro-Oncology. 2015;17(suppl_2):ii3–ii8. doi:10.1093/neuonc/nou354
59. Pawar S, Koneru T, McCord E, Tatiparti K, Sau S, Iyer AK. LDL receptors and their role in targeted therapy for glioma: a review. Drug Discovery Today. 2021;26(5):1212–1225. doi:10.1016/j.drudis.2021.02.008
60. Begines B, Ortiz T, Pérez-Aranda M, et al. Polymeric nanoparticles for drug delivery: recent developments and future prospects. Nanomaterials. 2020;10(7):1403. doi:10.3390/nano10071403
61. Locatelli E, Comes Franchini M. Biodegradable PLGA-b-PEG polymeric nanoparticles: synthesis, properties, and nanomedical applications as drug delivery system. J Nanopart Res. 2012;14(12):1316. doi:10.1007/s11051-012-1316-4
62. Fan S, Zheng Y, Liu X, et al. Curcumin-loaded PLGA-PEG nanoparticles conjugated with B6 peptide for potential use in Alzheimer’s disease. Drug Delivery. 2018;25(1):1091–1102. doi:10.1080/10717544.2018.1461955
63. Carradori D, Balducci C, Re F, et al. Antibody-functionalized polymer nanoparticle leading to memory recovery in Alzheimer’s disease-like transgenic mouse model. Nanomed Nanotechnol Biol Med. 2018;14(2):609–618. doi:10.1016/j.nano.2017.12.006
64. Brambilla D, Le Droumaguet B, Nicolas J, et al. Nanotechnologies for Alzheimer’s disease: diagnosis, therapy, and safety issues. Nanomed Nanotechnol Biol Med. 2011;7(5):521–540. doi:10.1016/j.nano.2011.03.008
65. Bozdağ Pehlivan S. Nanotechnology-based drug delivery systems for targeting, imaging and diagnosis of neurodegenerative diseases. Pharm Res. 2013;30(10):2499–2511. doi:10.1007/s11095-013-1156-7
66. Krol S, Macrez R, Docagne F, et al. Therapeutic benefits from nanoparticles: the potential significance of nanoscience in diseases with compromise to the blood brain barrier. Chem Rev. 2013;113(3):1877–1903. doi:10.1021/cr200472g
67. Kaur J, Gulati M, Kapoor B, et al. Advances in designing of polymeric micelles for biomedical application in brain related diseases. Chem Biol Interact. 2022;361:109960. doi:10.1016/j.cbi.2022.109960
68. Aguzzi A, Lakkaraju AKK, Frontzek K. Toward therapy of human prion diseases. Annu Rev Pharmacol Toxicol. 2018;58(1):331–351. doi:10.1146/annurev-pharmtox-010617-052745
69. Santos A, Veiga F, Figueiras A. Dendrimers as pharmaceutical excipients: synthesis, properties, toxicity and biomedical applications. Materials. 2020;13(1):65. doi:10.3390/ma13010065
70. Lee CC, MacKay JA, Fréchet JMJ, Szoka FC. Designing dendrimers for biological applications. Nat Biotechnol. 2005;23(12):1517–1526. doi:10.1038/nbt1171
71. Zhu W, Castro NJ, Shen YL, Zhang LG. Chapter 16 - Nanotechnology and 3D/4D Bioprinting for Neural Tissue Regeneration. In: Zhang LG, Fisher JP, Leong KW, editors. 3D Bioprinting and Nanotechnology in Tissue Engineering and Regenerative Medicine.
72. Lu Y, Rivera-Rodriguez A, Tay ZW, et al. Combining magnetic particle imaging and magnetic fluid hyperthermia for localized and image-guided treatment. Int j Hyperthermia. 2020;37(3):141–154. doi:10.1080/02656736.2020.1853252
73. Zhou H, Fan Z, Li PY, et al. Dense and dynamic polyethylene glycol shells cloak nanoparticles from uptake by liver endothelial cells for long blood circulation. ACS Nano. 2018;12(10):10130–10141. doi:10.1021/acsnano.8b04947
74. Rashwan AK, Karim N, Xu Y, et al. An updated and comprehensive review on the potential health effects of curcumin-encapsulated micro/nanoparticles. Crit Rev Food Sci Nutr. 2022;1(1):1–21. doi:10.1080/10408398.2022.2070906
75. Souri M, Soltani M, Moradi Kashkooli F, Kiani shahvandi M. Engineered strategies to enhance tumor penetration of drug-loaded nanoparticles. J Control Release. 2022;341:227–246. doi:10.1016/j.jconrel.2021.11.024
76. Peng L, Liang Y, Zhong X, et al. Aptamer-conjugated gold nanoparticles targeting epidermal growth factor receptor variant III for the treatment of glioblastoma. Int J Nanomed. 2020;15:1363–1372. doi:10.2147/IJN.S238206
77. Salameh JW, Zhou L, Ward SM, Santa Chalarca CF, Emrick T, Figueiredo ML. Polymer-mediated gene therapy: recent advances and merging of delivery techniques. WIREs Nanomed Nanobiotechnol. 2020;12(2):e1598. doi:10.1002/wnan.1598
78. Yi C, Yang Y, Liu B, He J, Nie Z. Polymer-guided assembly of inorganic nanoparticles. Chem Soc Rev. 2020;49(2):465–508. doi:10.1039/C9CS00725C
79. Karuppusamy C, Palanivel V. Role of nanoparticles in drug delivery system: a comprehensive review. Int J Med. 2017;9:318–325.
80. Adepu S, Ramakrishna S. Controlled drug delivery systems: current status and future directions. Molecules. 2021;26(19):5905. doi:10.3390/molecules26195905
81. Tang F, Li L, Chen D. Mesoporous silica nanoparticles: synthesis, biocompatibility and drug delivery. Adv Mater. 2012;24(12):1504–1534. doi:10.1002/adma.201104763
82. Yin Q, Shen J, Zhang Z, Yu H, Li Y. Reversal of multidrug resistance by stimuli-responsive drug delivery systems for therapy of tumor. Adv. Drug Delivery Rev. 2013;65(13):1699–1715. doi:10.1016/j.addr.2013.04.011
83. Nguyen CV, Ye Q, Meyyappan M. Carbon nanotube tips for scanning probe microscopy: fabrication and high aspect ratio nanometrology. Meas Sci Technol. 2005;16(11):2138. doi:10.1088/0957-0233/16/11/003
84. Klumpp C, Kostarelos K, Prato M, Bianco A. Functionalized carbon nanotubes as emerging nanovectors for the delivery of therapeutics. Biochimica et Biophysica Acta. 2006;1758(3):404–412. doi:10.1016/j.bbamem.2005.10.008
85. Ranjita Misra SSP, Sanjeeb K. Nanoparticles: a Boon to Drug Delivery, Therapeutics, Diagnostics and Imaging. In: Nanomedicine in Cancer. Jenny Stanford Publishing; 2017.
86. Soni S, Ruhela RK, Medhi B. Nanomedicine in Central Nervous System (CNS) disorders: a present and future prospective. Adv Pharm Bull. 2016;6(3):319–335. doi:10.15171/apb.2016.044
87. Gaur M, Misra C, Yadav AB, et al. Biomedical applications of carbon nanomaterials: fullerenes, quantum dots, nanotubes, nanofibers, and graphene. Materials. 2021;14(20):5978. doi:10.3390/ma14205978
88. Gao G, Ahn M, Cho WW, Kim BS, Cho DW. 3D printing of pharmaceutical application: drug screening and drug delivery. Pharmaceutics. 2021;13(9):1373. doi:10.3390/pharmaceutics13091373
89. Janzen D, Bakirci E, Wieland A, Martin C, Dalton PD, Villmann C. Cortical neurons form a functional neuronal network in a 3D printed reinforced matrix. Adv. Healthcare Mater. 2020;9(9):1901630. doi:10.1002/adhm.201901630
90. Ye W, Li H, Yu K, et al. 3D printing of gelatin methacrylate-based nerve guidance conduits with multiple channels. Mater Des. 2020;192:108757. doi:10.1016/j.matdes.2020.108757
91. Saylam E, Akkaya Y, Ilhan E, et al. Levodopa-Loaded 3D-printed poly (lactic) acid/chitosan neural tissue scaffold as a promising drug delivery system for the treatment of parkinson’s disease. Appl Sci. 2021;11(22):10727. doi:10.3390/app112210727
92. Perkušić M, Nižić Nodilo L, Ugrina I, et al. Chitosan-based thermogelling system for nose-to-brain donepezil delivery: optimising formulation properties and nasal deposition profile. Pharmaceutics. 2023;15(6):1660. doi:10.3390/pharmaceutics15061660
93. Fujimaki H, Uchida K, Inoue G, et al. Oriented collagen tubes combined with basic fibroblast growth factor promote peripheral nerve regeneration in a 15 mm sciatic nerve defect rat model. J Biomed Mater Res A. 2017;105(1):8–14. doi:10.1002/jbm.a.35866
94. Wu Z, Li Q, Xie S, Shan X, Cai Z. In vitro and in vivo biocompatibility evaluation of a 3D bioprinted gelatin-sodium alginate/rat Schwann-cell scaffold. Mater Sci Eng C Mater Biol Appl. 2020;109:110530. doi:10.1016/j.msec.2019.110530
95. Gu Q, Tomaskovic-Crook E, Lozano R, et al. Functional 3D neural mini-tissues from printed gel-based bioink and human neural stem cells. Adv Healthc Mater. 2016;5(12):1429–1438. doi:10.1002/adhm.201600095
96. Gander C, Shi K, Nokhodchi A, Lam M. A review of the Benefits 3D printing brings to patients with neurological diseases. Pharmaceutics. 2023;15(3):892. doi:10.3390/pharmaceutics15030892
97. Thomas M, Willerth SM. 3-D bioprinting of neural tissue for applications in cell therapy and drug screening. Front Bioeng Biotechnol. 2017;5:69. doi:10.3389/fbioe.2017.00069
98. Amiri E, Sanjarnia P, Sadri B, Jafarkhani S, Khakbiz M. Recent advances and future directions of 3D to 6D printing in brain cancer treatment and neural tissue engineering. Biomedical Materials. 2023;18. doi:10.1088/1748-605X/ace9a4
99. Kristiawan RB, Imaduddin F, Ariawan D, Arifin Z. A review on the fused deposition modeling (FDM) 3D printing: filament processing, materials, and printing parameters. Open Eng. 2021;11(1):639–649. doi:10.1515/eng-2021-0063
100. Zhu S, Zhang T, Zheng L, et al. Combination strategies to maximize the benefits of cancer immunotherapy. J Hematol Oncol. 2021;14(1):156. doi:10.1186/s13045-021-01164-5
101. Rana I, Oh J, Baig J, Moon JH, Son S, Nam J. Nanocarriers for cancer nano-immunotherapy. Drug Deliv Transl Res. 2023;13(7):1936–1954. doi:10.1007/s13346-022-01241-3
102. Bulcha JT, Wang Y, Ma H, Tai PWL, Gao G. Viral vector platforms within the gene therapy landscape. Sig Transduct Target Ther. 2021;6(1):1–24. doi:10.1038/s41392-021-00487-6
103. Smith MW, Gumbleton M. Endocytosis at the blood–brain barrier: from basic understanding to drug delivery strategies. J Drug Targeting. 2006;14(4):191–214. doi:10.1080/10611860600650086
104. Chang EL, Ting CY, Hsu PH, et al. Angiogenesis-targeting microbubbles combined with ultrasound-mediated gene therapy in brain tumors. J Control Release. 2017;255:164–175. doi:10.1016/j.jconrel.2017.04.010
105. IAW H, Lam PYP, Hui KM. Identification and characterization of novel human glioma-specific peptides to potentiate tumor-specific gene delivery. Human Gene Therapy. 2004;15(8):719–732. doi:10.1089/1043034041648372
106. Yahya EB, Alqadhi AM. Recent trends in cancer therapy: a review on the current state of gene delivery. Life Sci. 2021;269:119087. doi:10.1016/j.lfs.2021.119087
107. Unnisa A, Greig NH, Kamal MA. Nanotechnology-based gene therapy as a credible tool in the treatment of Alzheimer’s disease. Neural Regen Res. 2023;18(10):2127–2133. doi:10.4103/1673-5374.369096
108. Yu C, Li L, Hu P, et al. Recent advances in stimulus-responsive nanocarriers for gene therapy. Adv Sci. 2021;8(14):2100540. doi:10.1002/advs.202100540
109. Sandoz D, Boisvieux-Ulrich E, Laugier C, Brard E. Interactions of estradiol benzoate and progesterone on the development of the oviduct in quail. II. (Coturnix coturnix japonica). Ultrastructural studies of two types of responses obtained by varying doses of estradiol benzoate injected. Gen Comp Endocrinol. 1975;26(4):451–467. doi:10.1016/0016-6480(75)90168-9
110. Sudhakar V, Richardson RM. Gene therapy for neurodegenerative diseases. Neurotherapeutics. 2019;16(1):166–175. doi:10.1007/s13311-018-00694-0
111. Berndt P, Winkler L, Cording J, et al. Tight junction proteins at the blood-brain barrier: far more than claudin-5. Cell Mol Life Sci. 2019;76(10):1987–2002. doi:10.1007/s00018-019-03030-7
112. Negro S, Boeva L, Slowing K, Fernandez-Carballido A, Garcia-García L, Barcia E. Efficacy of ropinirole-loaded PLGA microspheres for the reversion of rotenone- induced parkinsonism. Curr Pharm Des. 2017;23(23):3423–3431. doi:10.2174/1381612822666160928145346
113. National Institute of Neurological Disorders and Stroke rt-PA Stroke Study Group. Tissue plasminogen activator for acute ischemic stroke. N Engl J Med. 1995;333(24):1581–1588. doi:10.1056/NEJM199512143332401
114. Celentano W, Pizzocri M, Moncalvo F, et al. Functional Poly(ε-caprolactone)/Poly(ethylene glycol) copolymers with complex topologies for doxorubicin delivery to a proteinase-rich tumor environment. ACS Appl Polym Mater. 2022;4(11):8043–8056. doi:10.1021/acsapm.2c00897
115. Han L, Jiang C. Evolution of blood–brain barrier in brain diseases and related systemic nanoscale brain-targeting drug delivery strategies. Acta Pharmaceutica Sinica B. 2021;11(8):2306–2325. doi:10.1016/j.apsb.2020.11.023
116. Zuardi AW, Crippa JS, Hallak JEC, Moreira FA, Guimarães FS. Cannabidiol, a Cannabis sativa constituent, as an antipsychotic drug. Braz J Med Biol Res. 2006;39:421–429. doi:10.1590/S0100-879X2006000400001
117. Kondiah PPD, Choonara YE, Kondiah PJ, et al. 17 - Nanocomposites for therapeutic application in multiple sclerosis. In: Inamuddin AAM, Mohammad A, editors. Applications of Nanocomposite Materials in Drug Delivery. Woodhead Publishing Series in Biomaterials. Woodhead Publishing; 2018:391–408. doi:10.1016/B978-0-12-813741-3.00017-0
118. Singh N, Miner A, Hennis L, Mittal S. Mechanisms of temozolomide resistance in glioblastoma - a comprehensive review. Cancer Drug Resist. 2021;4(1):17–43. doi:10.20517/cdr.2020.79
119. Teleanu RI, Preda MD, Niculescu AG, et al. Current strategies to enhance delivery of drugs across the blood-brain barrier. Pharmaceutics. 2022;14(5):987. doi:10.3390/pharmaceutics14050987
120. Barbara R, Belletti D, Pederzoli F, et al. Novel Curcumin loaded nanoparticles engineered for blood-brain barrier crossing and able to disrupt abeta aggregates. Int J Pharm. 2017;526(1–2):413–424. doi:10.1016/j.ijpharm.2017.05.015
121. Englert C, Trützschler AK, Raasch M, et al. Crossing the blood-brain barrier: glutathione-conjugated poly(ethylene imine) for gene delivery. J Control Release. 2016;241:1–14. doi:10.1016/j.jconrel.2016.08.039
122. Gao S, Tian H, Xing Z, et al. A non-viral suicide gene delivery system traversing the blood brain barrier for non-invasive glioma targeting treatment. J Control Release. 2016;243:357–369. doi:10.1016/j.jconrel.2016.10.027
123. O Q, M Y, Z W, T J, C Z, Z Q. New advances in brain-targeting nano-drug delivery systems for Alzheimer’s disease. J Drug Targeting. 2022;30(1). doi:10.1080/1061186X.2021.1927055
124. Rassu G, Soddu E, Posadino AM, et al. Nose-to-brain delivery of BACE1 siRNA loaded in solid lipid nanoparticles for Alzheimer’s therapy. Colloids Surf B Biointerfaces. 2017;152:296–301. doi:10.1016/j.colsurfb.2017.01.031
125. Ng EP, Mintova S. Nanoporous materials with enhanced hydrophilicity and high water sorption capacity. Microporous Mesoporous Mater. 2008;114(1):1–26. doi:10.1016/j.micromeso.2007.12.022
126. Niu S, Zhang LK, Zhang L, et al. Inhibition by multifunctional magnetic nanoparticles loaded with Alpha-Synuclein RNAi Plasmid in a parkinson’s disease model. Theranostics. 2017;7(2):344–356. doi:10.7150/thno.16562
127. Pan X, Veroniaina H, Su N, et al. Applications and developments of gene therapy drug delivery systems for genetic diseases. Asian J Pharm Sci. 2021;16(6):687–703. doi:10.1016/j.ajps.2021.05.003
128. Ishizu N, Yui D, Hebisawa A, et al. Impaired striatal dopamine release in homozygous Vps35 D620N knock-in mice. Hum Mol Genet. 2016;25(20):4507–4517. doi:10.1093/hmg/ddw279
129. Han L, Cai Q, Tian D, et al. Targeted drug delivery to ischemic stroke via chlorotoxin-anchored, lexiscan-loaded nanoparticles. Nanomedicine. 2016;12(7):1833–1842. doi:10.1016/j.nano.2016.03.005
130. He C, Hu Y, Yin L, Tang C, Yin C. Effects of particle size and surface charge on cellular uptake and biodistribution of polymeric nanoparticles. Biomaterials. 2010;31(13):3657–3666. doi:10.1016/j.biomaterials.2010.01.065
131. Sun T, Zhang YS, Pang B, Hyun DC, Yang M, Xia Y. Engineered nanoparticles for drug delivery in cancer therapy. Angew Chem Int Ed. 2014;53(46):12320–12364. doi:10.1002/anie.201403036
132. Xie J, Shen Z, Anraku Y, Kataoka K, Chen X. Nanomaterial-based blood-brain-barrier (BBB) crossing strategies. Biomaterials. 2019;224:119491. doi:10.1016/j.biomaterials.2019.119491
133. Bhaskar S, Tian F, Stoeger T, et al. Multifunctional Nanocarriers for diagnostics, drug delivery and targeted treatment across blood-brain barrier: perspectives on tracking and neuroimaging. Particle Fibre Toxicol. 2010;7(1):3. doi:10.1186/1743-8977-7-3
134. Pardeshi CV, Belgamwar VS. Direct nose to brain drug delivery via integrated nerve pathways bypassing the blood–brain barrier: an excellent platform for brain targeting. Expert Opin Drug Delivery. 2013;10(7):957–972. doi:10.1517/17425247.2013.790887
135. Graff CL, Pollack GM. Nasal drug administration: potential for targeted central nervous system delivery. J Pharmaceut Sci. 2005;94(6):1187–1195. doi:10.1002/jps.20318
136. Zhang X. Clinical Applications of Tumor-targeted Systems. In: Huang R, Wang Y editors. New Nanomaterials and Techniques for Tumor-Targeted Systems. Springer; 2020:437–456. doi:10.1007/978-981-15-5159-8_13.
137. Correia AC, Monteiro AR, Silva R, Moreira JN, Sousa Lobo JM, Silva AC. Lipid nanoparticles strategies to modify pharmacokinetics of central nervous system targeting drugs: crossing or circumventing the blood–brain barrier (BBB) to manage neurological disorders. Adv. Drug Delivery Rev. 2022;189:114485. doi:10.1016/j.addr.2022.114485
138. De Martini LB, Sulmona C, Brambilla L, Rossi D. Cell-penetrating peptides as valuable tools for nose-to-brain delivery of biological drugs. Cells. 2023;12(12):1643. doi:10.3390/cells12121643
139. Bhatia S. Nanoparticles Types, Classification, Characterization, Fabrication Methods and Drug Delivery Applications. In: Bhatia S editor. Natural Polymer Drug Delivery Systems: Nanoparticles, Plants, and Algae. Springer International Publishing; 2016:33–93. doi:10.1007/978-3-319-41129-3_2.
140. Tan Q, Zhao S, Xu T, et al. Getting drugs to the brain: advances and prospects of organic nanoparticle delivery systems for assisting drugs to cross the blood–brain barrier. J Mat Chem B. 2022;10(45):9314–9333. doi:10.1039/D2TB01440H
141. Kaur N, Kumar V, Mahender T, et al. Chapter 5 - Nanomedical drug delivery for neurodegenerative disease. In: Yadav AK, Shukla R, Flora SJS, editors. Nanomedical Drug Delivery for Neurodegenerative Diseases. Academic Press; 2022:67–79. doi:10.1016/B978-0-323-85544-0.00016-2
142. Bolhassani A. Potential efficacy of cell-penetrating peptides for nucleic acid and drug delivery in cancer. Biochimica et Biophysica Acta. 2011;1816(2):232–246. doi:10.1016/j.bbcan.2011.07.006
143. Fonseca SB, Pereira MP, Kelley SO. Recent advances in the use of cell-penetrating peptides for medical and biological applications. Adv. Drug Delivery Rev. 2009;61(11):953–964. doi:10.1016/j.addr.2009.06.001
144. Chugh A, Eudes F, Shim YS. Cell-penetrating peptides: nanocarrier for macromolecule delivery in living cells. IUBMB Life. 2010;62(3):183–193. doi:10.1002/iub.297
145. Hervé F, Ghinea N, Scherrmann JM. CNS delivery via adsorptive transcytosis. AAPS J. 2008;10(3):455–472. doi:10.1208/s12248-008-9055-2
146. Tashima T. Smart strategies for therapeutic agent delivery into brain across the blood–brain barrier using receptor-mediated transcytosis. Chem Pharm Bull. 2020;68(4):316–325. doi:10.1248/cpb.c19-00854
147. Toth AE, Holst MR, Nielsen MS. Vesicular transport machinery in brain endothelial cells: what we know and what we do not. Curr Pharm Des. 2020;26(13):1405–1416. doi:10.2174/1381612826666200212113421
148. Trompetero A, Gordillo A, Del Pilar MC, Cristina V, Bustos Cruz RH. Alzheimer’s disease and parkinson’s disease: a review of current treatment adopting a nanotechnology approach. Curr Pharm Des. 2018;24(1):22–45. doi:10.2174/1381612823666170828133059
149. Kadry H, Noorani B, Cucullo L. A blood-brain barrier overview on structure, function, impairment, and biomarkers of integrity. Fluids Barriers CNS. 2020;17(1):69. doi:10.1186/s12987-020-00230-3
150. Nguyen TT, Nguyen TTD, Tran NMA, Van Vo G. Lipid-based nanocarriers via nose-to-brain pathway for central nervous system disorders. Neurochem Res. 2022;47(3):552–573. doi:10.1007/s11064-021-03488-7
151. Pandit R, Chen L, Götz J. The blood-brain barrier: physiology and strategies for drug delivery. Adv. Drug Delivery Rev. 2020;165–166:1–14. doi:10.1016/j.addr.2019.11.009
152. Montegiove N, Calzoni E, Emiliani C, Cesaretti A. Biopolymer nanoparticles for nose-to-brain drug delivery: a new promising approach for the treatment of neurological diseases. J Functional Biomaterials. 2022;13(3):125. doi:10.3390/jfb13030125
153. Zha S, Wong KL, All AH. Intranasal delivery of functionalized polymeric nanomaterials to the brain. Adv Healthcare Mater. 2022;11(11):2102610. doi:10.1002/adhm.202102610
154. Xie A, Hanif S, Ouyang J, et al. Stimuli-responsive prodrug-based cancer nanomedicine. eBioMedicine. 2020:56. doi:10.1016/j.ebiom.2020.102821
155. Teleanu DM, Chircov C, Grumezescu AM, Teleanu RI. Neuronanomedicine: an up-to-date overview. Pharmaceutics. 2019;11(3):101. doi:10.3390/pharmaceutics11030101
156. Aryal M, Arvanitis CD, Alexander PM, McDannold N. Ultrasound-mediated blood–brain barrier disruption for targeted drug delivery in the central nervous system. Adv. Drug Delivery Rev. 2014;72:94–109. doi:10.1016/j.addr.2014.01.008
157. D’Agata F, Ruffinatti FA, Boschi S, et al. Magnetic nanoparticles in the central nervous system: targeting principles, applications and safety issues. Molecules. 2017;23(1):9. doi:10.3390/molecules23010009
158. Chatterjee DK, Fong LS, Zhang Y. Nanoparticles in photodynamic therapy: an emerging paradigm. Adv. Drug Delivery Rev. 2008;60(15):1627–1637. doi:10.1016/j.addr.2008.08.003
159. Rondon A, Mahri S, Morales-Yanez F, Dumoulin M, Vanbever R. Protein engineering strategies for improved pharmacokinetics. Adv Funct Mater. 2021;31(44):2101633. doi:10.1002/adfm.202101633
160. Arvizo RR, Bhattacharyya S, Kudgus RA, Giri K, Bhattacharya R, Mukherjee P. Intrinsic therapeutic applications of noble metal nanoparticles: past, present and future. Chem Soc Rev. 2012;41(7):2943–2970. doi:10.1039/C2CS15355F
161. Kim SS, Noh YH, Yoon OJ, Yoo SH. Carbon nanotubes serving as stem cell scaffold. Available from: https://patents.google.com/patent/US20090148417A1/en.
162. Chountoulesi M, Demetzos C. Promising nanotechnology approaches in treatment of autoimmune diseases of central nervous system. Brain Sci. 2020;10(6):338. doi:10.3390/brainsci10060338
163. Reddy JR. Preventive and therapeutic vaccine for Alzheimer’s disease. Available from: https://patents.google.com/patent/US20100173004A1/en.
164. Barenholz Y, Ovadia H, Kizelsztein P. Liposomal formulations comprising an amphipathic weak base like tempamine for treatment of neurodegenerative conditions. 2011. Available from: https://patents.google.com/patent/US20110027351A1/en.
165. Schwarz J, Weisspapir M. Colloidal solid lipid vehicle for pharmaceutical use. Available from: https://patents.google.com/patent/US20060222716A1/en.
166. Sung HW, Lin YH, Chen MC, Tu H. Nanoparticles for drug delivery. Available from: https://patents.google.com/patent/US20070237827A1/en.
167. Nelson T, Quattrone A, Alkon D. Artificial low-density lipoprotein carriers for transport of substances across the blood-brain barrier. Available from: https://patents.google.com/patent/US7682627B2/en.
168. Duskey JT, Belletti D, Pederzoli F, et al. Chapter One - Current Strategies for the Delivery of Therapeutic Proteins and Enzymes to Treat Brain Disorders. In: Sharma HS, Sharma A, editors. International Review of Neurobiology. Vol 137. Nanomedicine in Central Nervous System Injury and Repair. Academic Press; 2017:1–28. doi:10.1016/bs.irn.2017.08.006
169. Khafagy ES, Morishita M, Onuki Y, Takayama K. Current challenges in non-invasive insulin delivery systems: a comparative review. Adv Drug Delivery Rev. 2007;59(15):1521–1546. doi:10.1016/j.addr.2007.08.019
170. Gänger S, Schindowski K. Tailoring formulations for intranasal nose-to-brain delivery: a review on architecture, physico-chemical characteristics and mucociliary clearance of the nasal olfactory mucosa. Pharmaceutics. 2018;10(3):116. doi:10.3390/pharmaceutics10030116
171. Salunkhe S, Basak M, Chitkara D, Mittal A. Surface functionalization of exosomes for target-specific delivery and in vivo imaging & tracking: strategies and significance. J Control Release. 2020;326:599–614. doi:10.1016/j.jconrel.2020.07.042
172. Hinge NS, Kathuria H, Pandey MM. Engineering of structural and functional properties of nanotherapeutics and nanodiagnostics for intranasal brain targeting in Alzheimer’s. Appl Mater Today. 2022;26:101303. doi:10.1016/j.apmt.2021.101303
173. Weber WA, Czernin J, Anderson CJ, et al. The future of nuclear medicine, molecular imaging, and theranostics. J Nucl Med. 2020;61(Supplement 2):263S–272S. doi:10.2967/jnumed.120.254532
174. Chorny M, Hood E, Levy RJ, Muzykantov VR. Endothelial delivery of antioxidant enzymes loaded into non-polymeric magnetic nanoparticles. J Control Release. 2010;146(1):144–151. doi:10.1016/j.jconrel.2010.05.003
175. Galliani M, Santi M, Del Grosso A, et al. Cross-linked enzyme aggregates as versatile tool for enzyme delivery: application to polymeric nanoparticles. Bioconjugate Chem. 2018;29(7):2225–2231. doi:10.1021/acs.bioconjchem.8b00206
176. Hamill K. Delivery of an active lysosomal enzyme using GNeosomes. J Mat Chem B. 2016;4(35):5794–5797. doi:10.1039/C6TB01387B
177. Mukherjee S, Madamsetty VS, Bhattacharya D, Roy Chowdhury S, Paul MK, Mukherjee A. Recent advancements of nanomedicine in neurodegenerative disorders theranostics. Adv Funct Mater. 2020;30(35):2003054. doi:10.1002/adfm.202003054
178. Veiga N, Diesendruck Y, Peer D. Targeted nanomedicine: lessons learned and future directions. J Control Release. 2023;355:446–457. doi:10.1016/j.jconrel.2023.02.010
179. Lembo D, Donalisio M, Civra A, Argenziano M, Cavalli R. Nanomedicine formulations for the delivery of antiviral drugs: a promising solution for the treatment of viral infections. Expert Opin Drug Delivery. 2018;15(1):93–114. doi:10.1080/17425247.2017.1360863
180. Blau R, Krivitsky A, Epshtein Y, Satchi-Fainaro R. Are nanotheranostics and nanodiagnostics-guided drug delivery stepping stones towards precision medicine? Drug Resist Updates. 2016;27:39–58. doi:10.1016/j.drup.2016.06.003
181. Swift B, Jain L, White C, et al. Innovation at the intersection of clinical trials and real-world data science to advance patient care. Clin Transl Sci. 2018;11(5):450–460. doi:10.1111/cts.12559
182. Mura S, Couvreur P. Nanotheranostics for personalized medicine. Adv Drug Delivery Rev. 2012;64(13):1394–1416. doi:10.1016/j.addr.2012.06.006
183. Bhaladhare S, Bhattacharjee S. Chemical, physical, and biological stimuli-responsive nanogels for biomedical applications (mechanisms, concepts, and advancements): a review. Int J Biol Macromol. 2023;226:535–553. doi:10.1016/j.ijbiomac.2022.12.076
184. Kaushik A, Jayant RD, Bhardwaj V, Nair M. Personalized nanomedicine for CNS diseases. Drug Discovery Today. 2018;23(5):1007–1015. doi:10.1016/j.drudis.2017.11.010
185. Mishra AK, Das R, Sahoo S, Biswal B. Chapter Ten - Global regulations and legislations on nanoparticles usage and application in diverse horizons. In: Turan NB, Engin GO, Bilgili MS editors. Comprehensive Analytical Chemistry. Vol 99. Environmental Nanotechnology: Implications and Applications. Elsevier; 2022:261–290. doi:10.1016/bs.coac.2021.12.004.
186. Sztandera K, Gorzkiewicz M, Klajnert-Maculewicz B. Gold nanoparticles in cancer treatment. Mol Pharm. 2019;16(1):1–23. doi:10.1021/acs.molpharmaceut.8b00810
187. Bobo D, Robinson KJ, Islam J, Thurecht KJ, Corrie SR. Nanoparticle-based medicines: a review of FDA-approved materials and clinical trials to date. Pharm Res. 2016;33(10):2373–2387. doi:10.1007/s11095-016-1958-5
188. Foulkes R, Man E, Thind J, Yeung S, Joy A, Hoskins C. The regulation of nanomaterials and nanomedicines for clinical application: current and future perspectives. Biomater Sci. 2020;8(17):4653–4664. doi:10.1039/D0BM00558D
189. Alphandéry E, Grand-Dewyse P, Lefèvre R, Mandawala C, Durand-Dubief M. Cancer therapy using nanoformulated substances: scientific, regulatory and financial aspects. Expert Rev Anticancer Ther. 2015;15(10):1233–1255. doi:10.1586/14737140.2015.1086647
190. Bregoli L, Movia D, Gavigan-Imedio JD, Lysaght J, Reynolds J, Prina-Mello A. Nanomedicine applied to translational oncology: a future perspective on cancer treatment. Nanomed Nanotechnol Biol Med. 2016;12(1):81–103. doi:10.1016/j.nano.2015.08.006
191. Fan AM, Alexeeff G. Nanotechnology and nanomaterials: toxicology, risk assessment, and regulations. J Nanosci Nanotech. 2010;10(12):8646–8657. doi:10.1166/jnn.2010.2493
192. Luijten PR, van Dongen GAMS, Moonen CT, Storm G, Crommelin DJA. Public–private partnerships in translational medicine: concepts and practical examples. J Control Release. 2012;161(2):416–421. doi:10.1016/j.jconrel.2012.03.012
193. Bella D, Antonietta M. Overview and update on extracellular vesicles: considerations on exosomes and their application in modern medicine. Biology. 2022;11(6):804. doi:10.3390/biology11060804
194. R B, De JW, Re G Nanotechnology in medical applications: state-of-the-art in materials and devices. Rijksinstituut voor Volksgezondheid en Milieu RIVM; 2005. Available from: https://rivm.openrepository.com/handle/10029/7265.
195. George A, Shah PA, Shrivastav PS. Natural biodegradable polymers based nano-formulations for drug delivery: a review. Int J Pharm. 2019;561:244–264. doi:10.1016/j.ijpharm.2019.03.011
196. Wicki A, Witzigmann D, Balasubramanian V, Huwyler J. Nanomedicine in cancer therapy: challenges, opportunities, and clinical applications. J Control Release. 2015;200:138–157. doi:10.1016/j.jconrel.2014.12.030
197. Laurent S, Dutz S, Häfeli UO, Mahmoudi M. Magnetic fluid hyperthermia: focus on superparamagnetic iron oxide nanoparticles. Adv Colloid Interface Sci. 2011;166(1):8–23. doi:10.1016/j.cis.2011.04.003
198. Shah S, Famta P, Bagasariya D, et al. Tuning mesoporous silica nanoparticles in novel avenues of cancer therapy. Mol Pharm. 2022;19(12):4428–4452. doi:10.1021/acs.molpharmaceut.2c00374
199. Chandrakala V, Aruna V, Angajala G. Review on metal nanoparticles as nanocarriers: current challenges and perspectives in drug delivery systems. Emergent Mater. 2022;5(6):1593–1615. doi:10.1007/s42247-021-00335-x
200. Alshawwa SZ, Kassem AA, Farid RM, Mostafa SK, Labib GS. Nanocarrier drug delivery systems: characterization, limitations, future perspectives and implementation of artificial intelligence. Pharmaceutics. 2022;14(4):883. doi:10.3390/pharmaceutics14040883
201. Huynh L, Neale C, Pomès R, Allen C. Computational approaches to the rational design of nanoemulsions, polymeric micelles, and dendrimers for drug delivery. Nanomedicine. 2012;8(1):20–36. doi:10.1016/j.nano.2011.05.006
202. He Y, Ye Z, Liu X, et al. Can machine learning predict drug nanocrystals? J Control Release. 2020;322:274–285. doi:10.1016/j.jconrel.2020.03.043
203. Duarte Y, Márquez-Miranda V, Miossec MJ, González-Nilo F. Integration of target discovery, drug discovery and drug delivery: a review on computational strategies. Wiley Interdiscip Rev Nano Nanobio. 2019;11(4):e1554. doi:10.1002/wnan.1554
204. Gonzalez-Ibanez AM, Gonzalez-Nilo F, Cachau R. The collaboratory for structural nanobiology. Biophys J. 2009;96(3):49a. doi:10.1016/j.bpj.2008.12.151
205. Ostraat ML, Mills KC, Guzan KA, Murry D. The Nanomaterial Registry: facilitating the sharing and analysis of data in the diverse nanomaterial community. Int J Nanomed. 2013;8(sup1):7–13. doi:10.2147/IJN.S40722
© 2024 The Author(s). This work is published and licensed by Dove Medical Press Limited. The full terms of this license are available at https://www.dovepress.com/terms.php and incorporate the Creative Commons Attribution - Non Commercial (unported, v3.0) License.
By accessing the work you hereby accept the Terms. Non-commercial uses of the work are permitted without any further permission from Dove Medical Press Limited, provided the work is properly attributed. For permission for commercial use of this work, please see paragraphs 4.2 and 5 of our Terms.